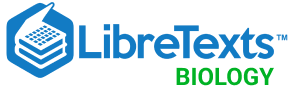
- school Campus Bookshelves
- menu_book Bookshelves
- perm_media Learning Objects
- login Login
- how_to_reg Request Instructor Account
- hub Instructor Commons
- Download Page (PDF)
- Download Full Book (PDF)
- Periodic Table
- Physics Constants
- Scientific Calculator
- Reference & Cite
- Tools expand_more
- Readability
selected template will load here
This action is not available.
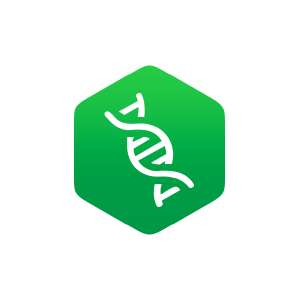

4.5.2: Translocation (Assimilate Transport)
- Last updated
- Save as PDF
- Page ID 32039
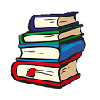
- Melissa Ha, Maria Morrow, & Kammy Algiers
- Yuba College, College of the Redwoods, & Ventura College via ASCCC Open Educational Resources Initiative
Learning Objectives
- Distinguish between sources and sinks and provide examples of each.
- Explain the process of phloem loading, distinguishing between apoplastic and symplastic pathways.
- Explain how assimilate translocations through the phloem according to the pressure-flow hypothesis.
Plants need an energy source to grow. In seeds and bulbs, food is stored in polymers (such as starch) that are converted by metabolic processes into sucrose for newly developing plants. Once green shoots and leaves are growing, plants are able to produce their own food through photosynthesis. The products of photosynthesis (mainly the sugar sucrose) are a major component of the substance found in the phloem, called assimilate . Ions, amino acids, certain hormones, and other molecules are also found in assimilate. The movement of assimilate is called translocation , or assimilate transport.
Sources and Sinks
Structures that produce or release sugars for the growing plant are referred to as sources . Examples include mature leaves, which produce sugar through photosynthesis, and storage organs, such bulbs, tubers, or storage roots. Sources produce or store more sugars than they need themselves and can thus export sugars. The points of sugar delivery, such as most roots, young shoots, and developing fruits and seeds, are called sinks (F igure \(\PageIndex{1}\)). Because sinks do not produce enough sugars to meet their energy needs, they must import sugars from sources.
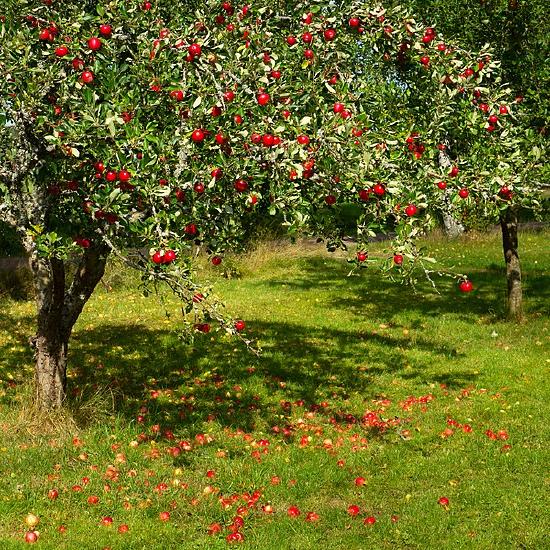
The pattern of assimilate flow changes as the plant grows and develops. Sugars are directed primarily to the roots early on, to shoots and leaves during vegetative growth, and to seeds and fruits during reproductive development. They are also directed to storage structures. Thus, the same organ may function as a source or a sink depending on the stage of development. For example, a young leaf may initially be a sink, but it will eventually grow and conduct enough photosynthesis to become a source. Similarly, a developing seed is a sink as the embryo develops. Once the seed germinates, however, starches stored in the seed break down, act as a source for the growing seedling structures.
The products from the source are usually translocated to the nearest sink through the phloem. For example, the highest leaves will send sugars upward to the growing shoot tip, whereas lower leaves will direct sugars downward to the roots. Intermediate leaves will send products in both directions, unlike the flow in the xylem, which is always unidirectional (soil to leaf to atmosphere).
Phloem Loading
Before discussing how sources transport sugars into the phloem ( phloem loading ), let's first review the conducting cells of the phloem. Stacks of these cylindrical cells called sieve-tube elements form column-like structures. Each cell is separated by a sieve plate (sieve-tube plate). The sieve plate has holes in it, like a slice of Swiss cheese, allowing for translocation. Lateral sieve areas on the side of the column allow different phloem tubes to interact. Sieve-tube elements have reduced cytoplasmic contents and rely on special parenchyma cells called companion cells, which assist with metabolic activities and provide energy (Figure \(\PageIndex{2}\)). In addition to sieve-tube elements and companion cells, the phloem contains other parenchyma cells and may contain sclerenchyma fibers. In leaves, the phloem is found in vascular bundles (leaf veins), which are surrounded by a bundle sheath. Sugars are produced through photosynthesis in the mesophyll cells that fill most of the leaf (see diagram in Transpiration section).
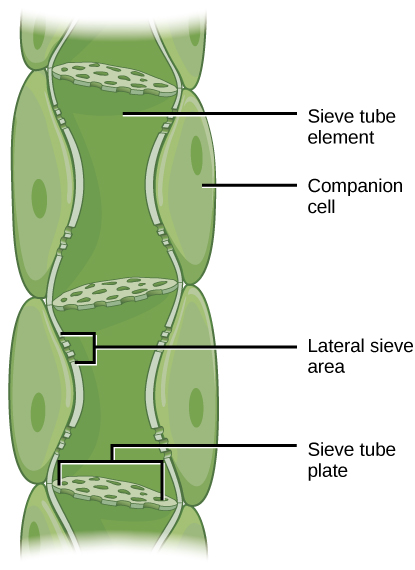
There are two pathways of phloem loading (Figure \(\PageIndex{3}\)). Both pathways begin the same way. The cytoplasms of most plant cells are interconnected via plasmodesmata.Augars through plasmodesmata from mesophyll cells to bundle sheath cells to the parenchyma cells of the phloem. From there, the pathways differ. The first pathway called apoplastic phloem loading , occurs when the regular parenchyma cells of phloem are not connected to the companion cells. In this case, sucrose must exist the regular parenchyma cells and enter the apoplast. It enters the companion cell cytoplasm as well as the inside of the sieve tube through secondary active transport . Protons are pumped into the apoplast (outside of the cells), when the protons re-enter the cells (move down their concentration gradient), they bring sucrose with them by flowing through carrier proteins called sucrose-proton symporters . In this way, sucrose is actively transported against its concentration gradient, and high sucrose concentrations accumulate in the assimilate of the phloem. The second pathway is symplastic phloem loading . When the regular parenchyma cells of the phloem are connected to companion cells via plamodesmata, sucrose can flow through the symplast all the way to the sieve-tube elements. There is no need for sucrose to leave the cytoplasm (enter the apoplast) and re-enter cells via sucrose-proton symport.
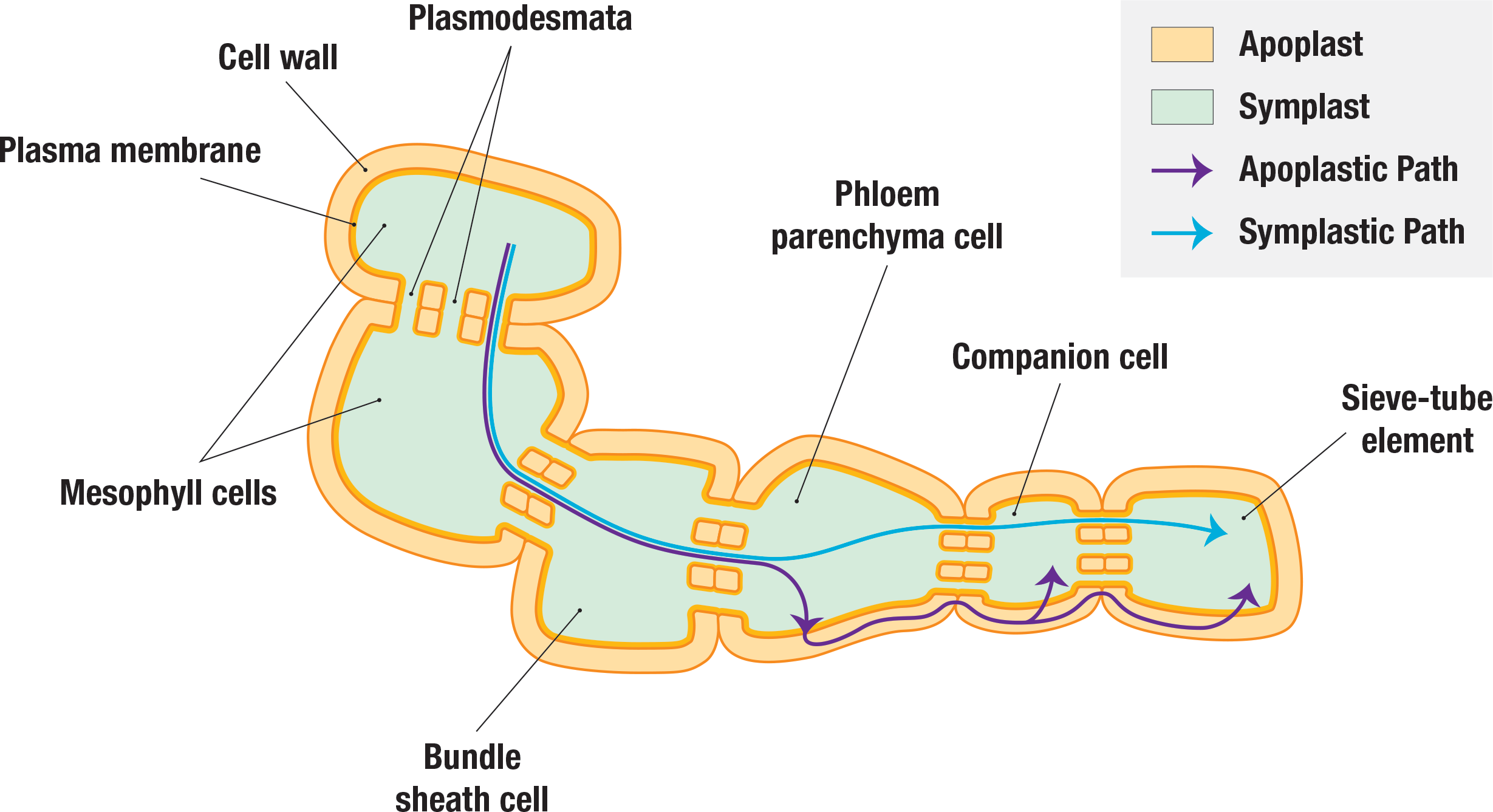
Pressure-Flow Hypothesis
What drives the movement of assimilate in the phloem? According to the pressure-flow hypothesis , assimilate moves due to osmosis of water to and from the xylem. While this is a passive process, it ultimately results from the active transport of sugars during phloem loading and unloading. In contrast with transpiration, an entirely passive process, translocation as a passive process that is indirectly driven by active processes.
Assimilate contains up to 30 percent sugar, and this high solute concentration decreases Ψ s , which decreases the total water potential. The sugar concentration of the assimilate near sinks, where phloem loading occurs, is highest. This causes water to move by osmosis from the adjacent xylem into sieve tubes, thereby increasing pressure. The increase in pressure increases in total water potential causes the bulk flow of phloem from source to sink (Figure \(\PageIndex{4}\)). Sugar concentration in the sink cells is lower than in the sieve-tube elements because the sink sucrose has been metabolized for growth, or converted to starch for storage or other polymers, such as cellulose, for structural integrity. Unloading at the sink end of the phloem tube occurs by either diffusion or active transport of sucrose molecules from an area of high concentration to one of low concentration. Unloading reduces the sugar concentration of assimilate near sinks, increasing water potential. As a result, water moves from the phloem by osmosis and is then transpired or recycled via the xylem back into the phloem sap.
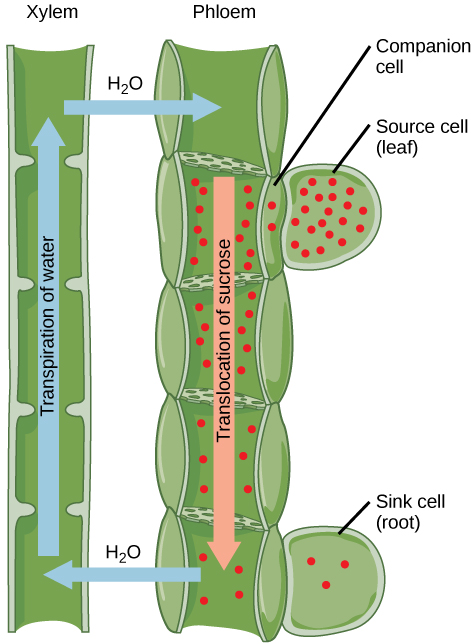
Attribution
Curated and authored by Melissa Ha using 30.5 Transport of Water and Solutes in Plants from Biology 2e by OpenStax (licensed CC-BY ). Access for free at openstax.org .
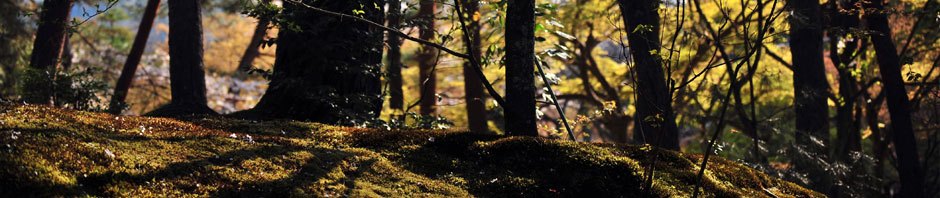
- About Organismal Biology
- Phylogenetic Trees and Geologic Time
- Prokaryotes: Bacteria & Archaea
- Eukaryotes and their Origins
- Land Plants
- Animals: Invertebrates
- Animals: Vertebrates
- The Tree of Life over Geologic Time
- Mass Extinctions and Climate Variability
- Multicellularity, Development, and Reproduction
- Animal Reproductive Strategies
- Animal Reproductive Structures and Functions
- Animal Development I: Fertilization & Cleavage
- Animal Development II: Gastrulation & Organogenesis
- Plant Reproduction
- Plant Development I: Tissue differentiation and function
- Plant Development II: Primary and Secondary Growth
- Principles of Chemical Signaling and Communication by Microbes
- Animal Hormones
- Plant Hormones and Sensory Systems
- Nervous Systems
- Animal Sensory Systems
- Motor proteins and muscles
- Motor units and skeletal systems
- Nutrient Needs and Adaptations
- Nutrient Acquisition by Plants
- Water Transport in Plants: Xylem
Sugar Transport in Plants: Phloem
- Nutrient Acquisition by Animals
- Animal Gas Exchange and Transport
- Animal Circulatory Systems
- The Mammalian Cardiac Cycle
- Ion and Water Regulation and Nitrogenous Wastes in Animals
- The Mammalian Kidney: How Nephrons Perform Osmoregulation
- Plant and Animal Responses to the Environment
Learning Objectives
- Identify examples of and differentiate between sugar sources and sugar sinks in plant tissues
- Explain the roles of solute potential, pressure potential, and movement of water in the Pressure Flow Model for sugar translocation in phloem tissue
- Describe the roles of proton pumps, co-transporters, and facilitated diffusion in the Pressure Flow Model
- Recognize that the transport pathway used to load sugars at sources or unload sugars at sinks will depend on whether sugar is moving down or against its concentration gradient
- Compare and contrast the mechanisms and requirements of fluid transport in xylem and phloem
Sugars Move from “Sources” to “Sinks”
The information below was adapted from OpenStax Biology 30.5
Plants need an energy source to grow. In growing plants, sugars move from sites where they are produced (or stored) to sites where they are needed for growth (or storage) via a process called translocation , or movement of sugar through the plant phloem:
- A source is any structure in a plant that either produces (like a leaf) or releases (like a storage bulb) sugars for the growing plant.
- A sink is any location where sugar is delivered for use in a growing tissue or storage for later use. Growing tissues might include apical and lateral meristems or developing leaves, flowers, seeds, and fruits; storage locations might include roots, tubers, and bulbs.
Notice that a storage location might be either a source or a sink, depending on the plant’s stage of development and the season:
- In the middle of the growing season , the sources include mature leaves and stems which are actively photosynthesizing and producing excess sugars. Sinks during the growing season include areas of active growth meristems, new leaves, and reproductive structures like flowers and seeds. Sinks also include sugar storage locations, such as roots, tubers, or bulbs.
- At the end of the growing season , the plant will drop leaves and no longer have actively photosynthesizing tissues. Depending on the local climate, the growing season may end either due to onset of winter or onset of the dry season.
- Early at the start of the next growing season , a plant must resume growth after dormancy. Because the plant has no existing leaves, its only source of sugar for growth is the sugar stored in roots, tubers, or bulbs from the last growing season. These storage sites now serve as sources, while actively developing leaves are sinks. Once the leaves mature, they will become sources of sugar during the growing season.
The Pressure Flow Model for Sugar Translocation Requires the Movement of Water into and out of the Phloem
The best-supported hypothesis to explain the movement of sugars in phloem is the pressure flow model for phloem transport. This hypothesis accounts for several observations:
- The fluid in phloem is under high positive pressure
- Translocation stops if the phloem tissue is killed
- Translocation can proceed in both directions simultaneously (but not within the same tube)
- Translocation is inhibited by compounds that stop ATP production in the sugar source
In very general terms, the pressure flow model works like this:
- A high concentration of sugar at the source creates a low solute potential (Ψs)
- The low solute potential draws water into the phloem from the adjacent xylem.
- Movement of water into the phloem creates a high pressure potential (Ψp), aka high turgor pressure, in the phloem.
- The high turgor pressure forces movement of phloem sap from source to sink through a process called “bulk flow.”
- The sugars moved via bulk flow are then rapidly removed from the phloem at the sink.
- Removal of the sugars at the sink then increases the Ψs, which causes water to leave the phloem and return to the xylem, which then decreases Ψp at the sink.
The video below provides a concise overview of sugar sources, sinks, and the pressure flow hypothesis:
Review of Transport Pathways in the Pressure Flow Model
Before we discuss more details of the pressure flow model, let’s first review some of the transport pathways we’ve previously discussed and add some new information to some of these transport pathways:
- Diffusion occurs when molecules move from an area of high concentration to an area of low concentration. Diffusion does not require energy because the molecules move down their concentration gradient (from areas of high to low concentration).
- Proton pumps use energy from ATP to create electrochemical gradients, with a high concentration of protons on one side of a plasma membrane. This electrochemical gradient can then be used as a source of energy to move other molecules against their concentration gradients via co-transporters.
- Symporters are a type of co-transporter that transports two molecules in the same direction; both into the cell, or both out of the cell.
- Antiporters are a type of co-transporter that transports two molecules in opposite directions; one into the cell, and the other out of the cell.
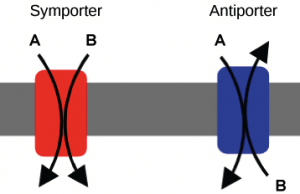
Transport Pathways in the Pressure Flow Model
Photosynthates such as sucrose (a type of sugar) are produced in parenchyma cells of photosynthesizing leaves. Sugars are actively transported (requires ATP) from source cells into the sieve-tube companion cells, which are associated with the sieve-tube elements in the vascular bundles. This active transport of sugar into the companion cells allows the companion cells to accumulate a higher concentration of sugar than is present in the photosynthesizing leaves. To make this process work, the companion cells use an ATP-powered proton pump to create an electrochemical gradient outside of the cell; then a proton-sucrose cotransporter couples the movement of a proton down its concentration gradient with sucrose against its concentration gradient and into the companion cells.
Once inside the companion cells, the sugar diffuses down its concentration gradient from the companion cell and into the phloem sieve-tube elements through the plasmodesmata that link the companion cell to the sieve tube elements. Recall that phloem sieve-tube elements have reduced cytoplasmic contents and are connected by pores that allow for pressure-driven bulk flow, or translocation, of phloem sap.
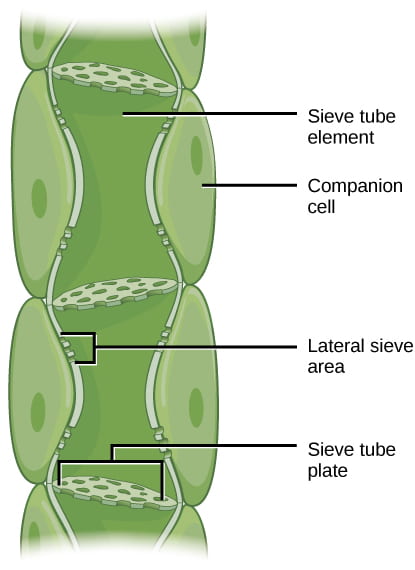
As noted in the previous section, the presence of high concentrations of sugar in the sieve tube elements drastically reduces Ψs; as a result, water then moves by osmosis from xylem into the phloem cells. This movement of water into the sieve tube cells then causes Ψp to increase, which increases both the turgor pressure in the phloem and the total water potential in the phloem at the source. This increase in water potential then drives the bulk flow of phloem from source to sink.
Unloading at the sink end of the phloem tube can occur either by diffusion , if the concentration of sucrose is lower at the sink than in the phloem, or by active transport , if the concentration of sucrose is higher at the sink than in the phloem:
- If the sink is an area of active growth, such as a new leaf or a reproductive structure, then the sucrose concentration in the sink cells is usually lower than in the phloem sieve-tube elements because the sink sucrose is rapidly metabolized for growth.
- If the sink is an area of storage where sugar is converted to starch, such as a root or bulb, then the sugar concentration in the sink is usually lower than in the phloem sieve-tube elements because the sink sucrose is rapidly converted to starch for storage.
- If the sink is an area of storage where the sugar is stored as sucrose, such as a sugar beet or sugar cane, then the sink may have a higher concentration of sugar than the phloem sieve-tube cells. In this situation, active transport by a proton-sucrose cotransporter (which relies on an ATP-powered proton pump) is typically used to transport sugar from the companion cells into storage vacuoles in the storage cells.
Once sugar is unloaded at the sink cells, the Ψs increases, causing water to diffuse by osmosis from the phloem back into the xylem. This movement of water out of the phloem causes Ψp to decrease, reducing the turgor pressure in the phloem at the sink and maintaining the direction of bulk flow from source to sink.

This video (beginning at 5:03) provides a more detailed discussion of the pressure flow hypothesis:
Movement of Fluid in Xylem vs Phloem
It should be clear that movement of sugars in phloem relies on the movement of water in phloem. But there are some important differences in the mechanisms of fluid movement in these two different vascular tissues:
- Xylem: transpiration (evaporation) from leaves, combined with cohesion and tension of water in the vessel elements and tracheids (passive; no energy required)
- Phloem: Active transport of sucrose from source cells into phloem sieve tube elements (energy required)
- Xylem: Non-living vessel elements and tracheids
- Phloem: Living sieve tube elements (supported by companion cells)
- Xylem: Negative due to pull from the top (Ψp decreases due to combined effects of transpiration combined with cohesion and tension of water)
- Phloem: Positive due to push from source (Ψp increases due to influx of water which increases turgor pressure at source)
- Entries RSS
- Comments RSS
- Sites@GeorgiaTech
Creative Commons License
Vannevar bush.
“Science has a simple faith, which transcends utility. It is the faith that it is the privilege of man to learn to understand, and that this is his mission.”
- Search Menu
- Advance articles
- Darwin Reviews
- Special Issues
- Expert View
- Flowering Newsletter Reviews
- Technical Innovations
- Editor's Choice
- Virtual Issues
- Community Resources
- Reasons to submit
- Author Guidelines
- Peer Reviewers
- Submission Site
- Open Access
- About Journal of Experimental Botany
- About the Society for Experimental Biology
- Editorial Board
- Advertising and Corporate Services
- Journals Career Network
- Permissions
- Self-Archiving Policy
- Dispatch Dates
- Journal metrics
- Journals on Oxford Academic
- Books on Oxford Academic
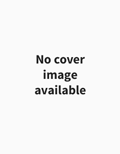
Article Contents
Introduction, münch theory and tall trees, three loading strategies and their ecophysiological function, leakage-retrieval mechanism, other phloem functions, conclusions, acknowledgements.
- < Previous
Phloem transport: a review of mechanisms and controls
- Article contents
- Figures & tables
- Supplementary Data
Veerle De Schepper, Tom De Swaef, Ingvar Bauweraerts, Kathy Steppe, Phloem transport: a review of mechanisms and controls, Journal of Experimental Botany , Volume 64, Issue 16, November 2013, Pages 4839–4850, https://doi.org/10.1093/jxb/ert302
- Permissions Icon Permissions
It is generally believed that an osmotically generated pressure gradient drives the phloem mass flow. So far, this widely accepted Münch theory has required remarkably few adaptations, but the debate on alternative and additional hypotheses is still ongoing. Recently, a possible shortcoming of the Münch theory has been pointed out, suggesting that the Münch pressure flow is more suitable for herbs than for trees. Estimation of the phloem resistance indicates that a point might be reached in long sieve tubes where the pressure required to drive the Münch flow cannot be generated. Therefore, the relay hypothesis regained belief as it implies that the sieve tubes are shorter then the plant’s axial axis. In the source phloem, three different loading strategies exist which probably result from evolutionary advantages. Passive diffusion seems to be the most primitive one, whereas active loading strategies substantially increase the growth potential. Along the transport phloem, a leakage-retrieval mechanism is observed. Appreciable amounts of carbohydrates are lost from the sieve tubes to feed the lateral sinks, while a part of these lost carbohydrates is subsequently reloaded into the sieve tubes. This mechanism is probably involved to buffer short-term irregularities in phloem turgor and gradient. In the long term, the mechanism controls the replenishment and remobilization of lateral stem storage tissues. As phloem of higher plants has multiple functions in plant development, reproduction, signalling, and growth, the fundamental understanding of the mechanisms behind phloem transport should be elucidated to increase our ability to influence plant growth and development.
The evolutionary journey of plants onto land involved the differentiation of the plant body into decentralized organs, such as leaves, roots, stem, and branches. These organs are interconnected at the whole-plant level by long-distance transport. Besides water, sugars are one of the most important components involved in this transport. The phloem tissue is the principal sugar conductive tissue in plants. Over 80 years ago, Ernest Münch (1930) proposed the now widely accepted mechanism for phloem transport. According to his theory, the mass flow in the phloem is driven by an osmotically generated pressure gradient. As the sieve pores interconnect the protoplasts of the sieve tubes, the transport in the sieve tube itself is a mass flow driven by a pressure (or turgor) gradient. Because the sieve tubes are separated by a plasma membrane from the surrounding plant cells, a higher solute concentration indirectly implies a higher turgor pressure as water will enter the sieve tubes by osmosis ( Gould et al. , 2005 ). The pressure gradient in the sieve tubes is generated by the accumulation (loading) of sugars and other osmotic substances at the sources and by their release (unloading) at the sinks ( Fig. 1 ). The sources are mainly leaves, whereas all energy-demanding or storage tissues are sinks (e.g. roots, fruits, and meristematic tissues).

A dynamic version of Münch’s pressure flow model, the local photoassimilates (violet arrows) and water (blue arrows), and the relative proportion of sieve elements (SEs) and companion cells (CCs) in the respective phloem zones. Photoassimilates are translocated via the phloem through essentially leaky instead of hermetically sealed pipes. The solute concentration, and implicitly the turgor, are controlled by release/retrieval mechanisms in the sieve element–companion cell complexes (SECCCs). Differential release/retrieval balances control the influx/efflux of sugars (violet arrows) and water (blue arrows) in the various phloem zones. In the collection phloem (phloem loading), the uptake or retrieval dominates; in the release phloem (phloem unloading), the release dominates. In the transport phloem, having a dual task (nourishment of lateral and terminal sinks), the balance between release and retrieval varies with the requirements of the plant. The gradual loss of solutes and commensurate amounts of water towards the sink, where massive delivery of water and solutes takes place, has been ascribed to the relative size reduction of CCs along the source to sink path, which may explain a decreasing retrieval capacity of the SECCCs in the direction of the sink ( van Bel, 2003 b . The phloem, a miracle of ingenuity. Plant, Cell and Environment 26, 125–149 with permission © John Wiley & Sons Ltd; Reprinted from Vascular transport in plants . van Bel and Hafke, 2005 . Physiological determinants of phloem transport. In: Holbrook NM, Zwieniecki MA, eds. Burlington: Elsevier, 19–44, with permission from Elsevier).
Along the phloem pathway, three successive functional sections can be defined, each with a specific task ( Van Bel and Hafke, 2005 ): collection, transport, and release ( Fig. 1 ). In the collection phloem, assimilates are loaded into the sieve element–companion cell complex (SECCC) of the minor leaf veins after being produced in the leaf mesophyll. Next, assimilates are transported towards the sinks via the transport phloem. The transport phloem is located in the major veins, petioles, branches, stem, and roots. The transport phloem has a dual function: it transports assimilates not only to terminal sinks (e.g. the roots and shoots) but also to lateral sinks along the path (e.g. the cambium) which are responsible for growth and tissue maintenance. These lateral sinks support the continuous leakage and retrieval of solutes along the pathway. The main task of the release phloem in the sinks is to unload assimilates from the SECCCs into growing or storage cells. The decreasing volume ratios between the companion cells and the sieve elements along the phloem stretch ( Fig. 1 ) may be related to a decreasing energy requirement for assimilate retention in collection, transport, and release phloem ( van Bel, 1996 , 2003 b ).
Over time, the original Münch theory has required remarkably few adaptations ( Thorpe et al. , 2005 ). It is generally believed that loading and unloading sites are both essential to maintain the osmotic gradient and control the rate of phloem translocations ( Gamalei, 2002 ). The loading and unloading mechanisms can be active or passive, and apoplastic (the sugars enter the apoplast at least once by crossing the cell membrane) or symplastic (entirely through the plasmodesmata-connected cytosol of cells) ( Turgeon, 2010 b ). The apoplast refers to the continuous system of cell walls and xylem vessels. In Münch’s original theory, the loading and unloading processes only occur in the sources (collection phloem) and sinks (release phloem), respectively. However, loading and unloading also take place along the phloem pathway (transport phloem) ( van Bel, 2003 a ; Thorpe et al. , 2005 ). This is called the leakage-retrieval mechanism. As such, the sieve tubes are considered as permeable instead of impermeable pipes. Recently, Thompson (2006) hypothesized that the pressure gradients along the phloem pathway should be low or negligible in order to regulate easily the solute exchange between the SECCCs and the surrounding tissues. His hypothesis, based on a detailed model study ( Thompson and Holbrook, 2004 ), seems physiologically acceptable because in the decentralized plant body, without a nervous system, individual cells can only sense and modify their own turgor and not the pressure gradients between them. Hence, according to this hypothesis, the transport phloem regulates the phloem turgor rather than the turgor gradient ( Thompson, 2006 ). In addition to this hypothesis, several ‘alternative’ hypotheses are still under debate. The most recent findings and questioned hypotheses are reviewed herein.
Recently, a possible shortcoming of Münch’s theory, especially in large trees, has been pointed out. According to Münch’s theory, the phloem flow ( F Pc ) is defined by the pressure ( P ) difference between sources and sinks and influenced by the resistance of the phloem pathway ( R Pc ):
Several experimental studies ( Fisher, 1978 ; Köckenberger et al. , 1997 ; Jahnke et al. , 1998 ; Windt et al. , 2006 ; De Schepper et al. , 2013 ) showed that phloem speed (proportional to F Pc ) across a wide range of angiosperm plants is roughly within the same range, namely around 1cm min –1 . Windt et al. (2006) hypothesized that the phloem is scaled and regulated to maintain a constant and relatively slow flow. In angiosperm trees the phloem speed seems to increase with tree height ( Dannoura et al. , 2011 ). Via a theoretical study, Thompson (2006) added that the pressure gradient along the phloem pathway ( P source – P sink ) should be low or negligible. Furthermore, Thompson and Holbrook (2003 a ) stated that the transit time of sucrose through a sieve tube is inversely proportional to the square of the sieve tube’s length. The phloem resistance ( R Pc ) in their model was mainly determined by the considered sieve plates whose number increased with the number of sieve elements and, thus, with the length of the sieve tube. Taking these findings into account, a contradiction arises for large plants, including trees. Because sources and sinks are symplastically connected according to Münch’s theory (1930) , it is generally assumed that the sieve tube’s length equals the plant’s axial length. In large trees, this implies that the phloem resistance becomes very large. According to Equation 1, a high phloem resistance ( R Pc ) results either in a large pressure difference ( P source – P sink ) or in a low phloem velocity ( F Pc ), which contradicts the above-mentioned findings. According to Münch’s theory, the phloem pressure in trees should be higher than those of herbaceous species since the required pressure difference increases with the resistance of the sieve tubes and, hence, the plant’s length. However, literature data suggest the opposite as the lowest measured values of phloem pressure are found in trees ( Hammel, 1968 ; Wright and Fisher, 1980 ; Sovonick-Dunford et al. , 1981 ; Pritchard, 2007 ). As such, phloem pressure does not scale to plant size ( Turgeon, 2010 a ). Moreover, estimations of phloem resistance based on the detailed architecture of sieve plates suggest that in long sieve tubes (as in large trees), a point might be reached where the pressure gradient required to drive the Münch flow exceeds the turgor pressure that can be generated ( Mullendore et al. , 2010 ). As such, the Münch pressure flow seems more suitable for herbs than for trees ( Turgeon, 2010 a ).
Based on these contradictions, several studies ( Thompson, 2006 ; Hölttä et al. , 2009 ; Jensen et al. , 2009 ; Knoblauch and Peters, 2010 ; Mullendore et al. , 2010 ; Turgeon, 2010 a ) suggested that, at least in some cases (e.g. large trees), the sieve tubes are shorter than the plant’s axial length. The proposed translocation pathway would be composed of series of shorter, overlapping sieve tubes with apoplastic loading steps between them. Lang (1979) called these intervening loading steps ‘relays’. In this relay system, solutes are energetically transported from one unit to the next, providing a boost in pressure at the relays along the transport pathway. The relay hypothesis does not conflict with Münch’s vision of phloem transport: the osmotically generated pressure flow is still the main mechanism of moving solutes between sites of active transport, but the speed and direction are additionally controlled near the relays ( Thompson and Holbrook, 2003 a ). However, the difficulty with the relay hypothesis is that phloem sap is rich in organic molecules and ions ( Turgeon, 2010 a ). Therefore, transfer across the plasma membrane requires an elaborated set of transporters unless the composition of the sap changes at each step ( Knoblauch and Peters, 2010 ; Turgeon, 2010 a ). Nevertheless, the leakage-retrieval process in the transport phloem is in favour of the relay theory ( Willenbrink, 2002 ; Thompson, 2006 ; Knoblauch and Peters, 2010 ). This leakage-retrieval mechanism, which is further discussed in a separate section, is interpreted by van Bel (2003 b , 2005 ) as a dynamic relay mechanism. However, the only experimental study that tested the relay hypothesis in castor bean ( Phaseolus vulgaris ) favoured hydrostatic continuity ( Murphy and Aikman, 1989 ), and the anatomical data currently available do not support the relay hypothesis.
A recent study ( Jensen et al. , 2011 , 2012 ) pointed out that, in addition to sieve tube conductivity, phloem speed is strongly influenced by the osmotic resistances present in the collection and release phloem. Most phloem studies (e.g. Thompson and Holbrook, 2003 a ) neglect these osmotic resistances through surface area. In contrast to the resistance in the transport phloem which is proportional to the plant length, the resistances in the collection and release phloem are inversely proportional to the source (e.g. leaf length) and sink (e.g. root length) length. By taking into account these resistances, a universal scaling law for phloem dimensions was discovered, namely the third power of the sieve tube radius linearly relates to leaf length multiplied by stem length. Because this relationship applies for all studied vascular plants (herbs and trees), it seems that all plants employ the same basic mechanism for an optimal phloem transport. As such, active transport facilitation, such as the relay mechanism, seems unlikely, because this would have altered the observed relationship in this study ( Jensen et al. , 2011 , 2012 ).
Table 1 summarizes the major differences regarding phloem transport between herbaceous plants and trees based on hypotheses and data found in the available literature. In addition, differences exist between angiosperm and gymnosperm trees due to their different phloem anatomy.
Phloem-related characteristics which differ between herbs and trees
* Hypotheses which are not yet experimentally proven.
Phloem loading is the starting point for the long-distance sugar transport: the accumulation of sugars osmotically increases the hydrostatic pressure in the sieve tubes. The route of sugar movement from mesophyll cells to sieve elements can be apoplastic or symplastic. The symplastic mode does not involve crossing the plasma membrane, while the apoplastic mode does. Besides the symplastic–apoplastic discrepancy, a distinction can be made between active and passive loading. In active loading, metabolic energy is used to pump assimilates into the phloem against a concentration gradient. In this view, three types or strategies of phloem loading can be defined in the collection phloem of the minor veins: active apoplastic loading, active symplastic polymer trapping, and passive symplastic diffusion ( Table 2 ). Each loading type corresponds to a specific type of companion cell in the SECCCs ( van Bel, 2003 b ; Van Bel and Hafke, 2005 ) ( Table 2 ).
During the process of active apoplastic loading, the sucrose destined for export enters the apoplast at some point and can only be pumped into the phloem from the apoplast by a carrier that is specific for sucrose ( Lalonde et al. , 2003 ; Van Bel and Hafke, 2005 ; Pittermann, 2010 ). The apoplastic loading is driven by a proton-motive force, maintained by proton pumps, and sucrose carriers (e.g. sucrose-symporter SUT4) in the plasma membrane of the SECCCs. Because of this active mechanism, a steep osmotic gradient can be created, which requires a commensurate influx of water, generating a high local turgor in the sieve tubes ( Van Bel and Hafke, 2005 ). The companion cell, specialized in apoplastic loading, is called a transfer cell and is characterized by (i) many cell wall invaginations to increase the plasma membrane surface and (ii) the presence of few plasmodesmata which connect with the mesophyll cell. Hence, the mesophyll and the SECCC operate virtually uncoupled as the symplastic connectivity between both is largely reduced ( van Bel, 2003 b ).
Polymer trapping occurs in species that translocate the raffinose family of the oligosaccharides. In a first step, assimilates synthesized in the mesophyll cells passively diffuse from the bundle sheath cells through abundant plasmodesmata into special companion cells, which are called intermediary cells. In these intermediary cells, the diffused assimilates are converted into the larger oligosaccharides, raffinose and stachyose (polymers made of three or four hexose sugars) ( Lalonde et al. , 2003 ; Turgeon and Ayre, 2005 ). A fundamental feature of this mechanism is that the branched plasmodesmata between the intermediary cells and the mesophyll cells are slightly narrower than those at many other interfaces. The size exclusion limits of these plasmodesmata allow diffusion of sucrose into the intermediary cell, but do not permit diffusion of the larger sugars, raffinose and stachyose, in the opposite direction. Hence, raffinose and stachyose accumulate in high concentrations in the SECCCs and sucrose continues to diffuse passively from the mesophyll cells, where it is synthesized, into the intermediary cells, where it is utilized ( Lalonde et al. , 2003 ; Turgeon and Ayre, 2005 ). Polymer trapping is an active mechanism, although it does not involve the active transport in the formal sense of moving ions and molecules across a membrane. It is thermodynamically active since energy is used to create a concentration difference between the mesophyll cells and the SECCCs ( Turgeon, 2010 b ).
Just recently, passive loading by diffusion has been recognized as a valuable loading strategy ( Turgeon, 2010 b ). Recent data ( Rennie and Turgeon, 2009 ) indicate that a large number of woody species, especially trees, load assimilates passively by maintaining high sucrose concentrations, and in some cases sugar alcohols, in the mesophyll cells. By definition, passive loading requires no energy input and is energetically downhill, with the sugar levels being higher in the mesophyll than in the phloem. Ions and molecules diffuse through plasmodesmata at each interface, without a concentrating step. The driving force for transport comes from the creation of a high solute concentration in the photosynthetic cells. These solutes diffuse from ordinary companion cells into the SECCCs through regularly appearing plasmodesmata. The corresponding hydrostatic pressure, engendered by the solutes in the sieve elements of the source phloem, sustains mass flow (not diffusion) towards the sinks ( Turgeon, 2010 b ). In this loading strategy, passive transport through plasmodesmata is referred to as diffusion, although a bulk flow may also be possible in plasmodesmata with sufficiently large radii ( Fisher and Cash-Clark, 2000 ). In contrast to active loading, passive loading is not accompanied by an uphill sucrose gradient from mesophyll to phloem. As such, this passive loading strategy can explain why the phloem osmotic potential and turgor pressure is low in trees ( Turgeon, 2010 a ). Passive loading has almost exclusively been observed in trees, making it difficult to argue that active loading is essential for efficient phloem transport. This is in agreement with Münch’s original theory which did not consider active loading ( Turgeon, 2010 b ).
Evaluation of the different loading strategies in an ecophysiological context revealed their evolutionary advantage ( Pritchard, 2007 ; Turgeon, 2010 a , b ). Active loading increases the efficiency to move a large amount of assimilates because the higher level of soluble carbohydrates in the sieve tubes maximizes the amount of carbon and energy delivered per unit of volume ( Turgeon, 2010 a ). Furthermore, active loading reduces the amount of non-structural carbohydrates (NSCs) in the leaf mesophyll without compromising export. Several studies ( Harper, 1989 ; Turgeon, 2010 b ) suggested that reducing non-productive carbon in the leaves significantly increases the plant growth potential. Another possible advantage of a low sugar concentration in the leaf mesophyll is the possible reduction of the feedback inhibition of photosynthesis ( Turgeon, 2010 b ). Therefore, active loading strategies substantially increase the growth potential of plants as they decrease the unnecessary inventory of NSCs in the leaves ( Turgeon, 2010 b ). Active loading is especially desired in herbaceous species, as their growth is rapid and their leaf production continuous (progressive addition of new leaves throughout the growing season). In trees, leaf production mostly occurs in periodic flushes, and much of the carbon used to fuel these flushes comes from storage. Therefore, the advantages of active loading are less important for trees, which often have relative growth rates well below that of herbs. Measured pre-dawn NSC levels in leaves confirm this hypothesis: the NSC levels of herbaceous crop plants are in general lower than those of woody plants ( Rennie and Turgeon, 2009 ). If this is true, storage of extra foliar NSC reserves in leaves of herbs can be considered as a safety factor, which becomes redundant in cultivated conditions. Hence, manipulation of storage in leaves could result in significant increases in growth potential of crop plants ( Turgeon, 2010 b ). It seems that the passive symplastic loading mode is the most primitive loading system, which evolved to active symplastic and apoplastic loading depending on the species requirements and growth conditions ( Lambers et al. , 1998 ).
Loading plays a crucial role in controlling the phloem hydrostatic pressure. In the collection phloem, loading can be regulated at two levels: at the level of leaf mesophyll cells or at the SECCC level. First, in the mesophyll, photosynthesis is the main process which indirectly controls loading as it determines the carbohydrate availability for export. The sugar concentration in the mesophyll cells directly influences the diffusion that is essential for symplastic loading. Regarding apoplastic loading, the mesophyll (apoplastic) solute concentration seems to control the sucrose carrier activity ( Barker et al. , 2000 ; Komor, 2000 ; Patrick et al. , 2001 ). Plants can control this carbohydrate availability for export as a sugar excess down-regulates photosynthesis by feedback mechanisms ( Foyer, 1988 ; Lewis et al. , 2000 ; Cheng et al. , 2008 ). These feedback mechanisms mostly prevent overproduction of photoassimilates, thus keeping supply to the SECCCs within appropriate limits ( Van Bel and Hafke, 2005 ). Secondly, phloem loading seems to be controlled by three state variables in the SECCCs: (i) the turgor pressure; (ii) the sucrose level; and (iii) the presence of phytohormones. Orlich et al. (1998) hypothesized that the SECCC turgor regulates the conductance of the plasmodesmata leading into the companion cells ( Turgeon and Ayre, 2005 ), as such influencing the symplastic loading. In addition, the SECCC turgor regulates the H + -ATPase activity which influences the proton-motive force that drives the sucrose carriers needed for apoplastic loading ( Daie, 1989 ; Patrick et al. , 2001 ; Lalonde et al. , 2003 ). The sucrose level in the SECCCs directly controls the diffusion of symplastic loading, while the apoplastic sucrose level regulates the activity of the apoplastic sucrose carriers ( Patrick et al. , 2001 ; Vaughn et al. , 2002 ; Lalonde et al. , 2003 ). High turgor and/or sucrose levels in the SECCC will decrease phloem loading. In addition, phytohormones can up- or down-regulate phloem loading ( Lalonde et al. , 1999 , 2003 ). Possible loading-regulating phytohormones are cytokinins, abscisic acid, auxins, and gibberellins ( Lalonde et al. , 2003 ).
Relationship between the phloem loading strategy at the minor vein and the ultrastructure of the companion cell (i) When apoplastic loading occurs, sucrose is pumped from the apoplast into the transfer cells (TCs) of the minor vein phloem by transporters (yellow circle), which enables the leaf to minimize the overall sucrose concentration in the leaf blade. Transfer cells (TCs) possess cell wall invaginations to increase the plasma membrane surface and have virtually no plasmodesmata at the MC–CC interface. (ii) When polymer trapping takes place, sucrose diffuses through plasmodesmata into the minor vein intermediary cells (ICs) and is converted to oligosaccharides, thus actively elevating the transport sugar concentration gradient in the phloem. ICs are characterized by numerous cytoplasmic vesicles and many plasmodesmata at the wall interface between mesophyll (MCs) and companion cells (CCs). (iii) If passive diffusion takes place, assimilates passively diffuse through plasmodesmata into simple companion cells (SCs) of the minor vein. The assimilate concentration in MCs, and therefore in the entire leaf, is high; the assimilate concentration in the veins is slightly lower. SCs, without particular properties, have a moderate plasmodesmal density at the MC–CC interface (adapted from van Bel, 2003 b . The phloem, a miracle of ingenuity. Plant, Cell and Environment 26, 125–149 with permission © John Wiley & Sons Ltd; Turgeon 2010 b . The role of phloem loading reconsidered. Plant Physiology 152, 1817–1823. www.plantphysiol.org , Copyright American Society of Plant Biologists).
The major function of the transport phloem is the translocation of carbohydrates from sources to sinks. While sources are specific tissues in which photosynthesis or remobilization takes place, sinks are present everywhere since maintenance respiration takes place in all living cells. Also more demanding sinks exist, needing a larger carbon influx to sustain growth (e.g. cambium) or storage (e.g. roots) ( Thorpe et al. , 2005 ). Hence, the transport phloem nourishes not only the terminal sinks but also many lateral sinks along the plant axis ( van Bel, 2003c ; Thorpe et al. , 2005 ; Van Bel and Hafke, 2005 ). To feed the lateral sinks, appreciable amounts of carbohydrates are lost from the SECCCs along the phloem pathway. However, a part of these lost carbohydrates is subsequently reloaded, or retrieved, into the SECCCs. This behaviour is called the leakage-retrieval mechanism and has been demonstrated in several studies using radioactively labelled assimilates ( Thorpe and Lang, 1983 ; Minchin and Thorpe, 1987 ; Minchin et al. , 2002 ; Gould et al. , 2004 ; Thorpe et al. , 2005 ; De Schepper et al. , 2013 ). This flexible mechanism allows rapid restoration of any osmotic disturbance along the pathway and, hence, locally buffers short-term irregularities in the turgor potential and gradient in the SECCCs ( Gould et al. , 2004 ; Van Bel and Hafke, 2005 ). These irregularities can be induced by temporal changes in source and/or sink activities. The leakage-retrieval mechanism controls the pressure gradient between the sources and the sinks to allow a constant flow between them and, as such, it decouples the activity of the sources and sinks in the short term ( Thorpe et al. , 2005 ).
The leakage-retrieval mechanism is based on a balance between leakage (or unloading) of carbohydrates out of the SECCCs into the surrounding apoplast and their subsequent retrieval (or reloading) ( Thorpe et al. , 2005 ). Today, most of the evidence indicates that the leakage mechanism follows an apoplastic route and is a passive diffusion process in which carbohydrates diffuse out of the SECCC symplast into the phloem apoplast ( Patrick et al. , 2001 ; Thorpe et al. , 2005 ). Under some specific conditions, such as high source–sink ratios, the leakage mechanism can change to a symplastic route ( Patrick and Offler, 1996 ; Patrick et al. , 2001 ). The SECCCs in the transport phloem are, under normal conditions, symplastically isolated from the surrounding tissues due to few, virtually closed plasmodesmata between the SECCCs and the phloem parenchyma cells ( van Bel, 2003 b , c ). These plasmodesmata can open under sink-limiting conditions, allowing symplastic leakage and, hence, the storage of the excess assimilates in the stem parenchyma ( Patrick and Offler, 1996 ; Patrick et al. , 2001 ; van Bel, 2003 c ). Unlike the leakage process, the mechanism of the retrieval process is active and mediated by sucrose symporters (e.g. SUT1 and SUC2) ( Patrick et al. , 2001 ). These symporters can be located at the plasma membrane of both the companion cell and the sieve element ( Kuhn et al. , 1997 ; Thorpe et al. , 2005 ). Due to their different mechanisms, the leakage and retrieval processes are controlled in a different way. The leakage diffusion process is mainly controlled by the symplastic SECCC assimilate concentration, as the apoplastic concentration is very low and changes in it will have little effect on the leakage diffusion ( Patrick, 1990 ; Thorpe et al. , 2005 ). The retrieval process, depending on sucrose carriers, will, in contrast, be sensitive to the apoplastic sucrose concentration and to the SECCC turgor ( Patrick et al. , 2001 ; Thorpe et al. , 2005 ). Furthermore, the apoplastic retrieval can be influenced by pH, potassium (K + ), and calcium (Ca 2+ ) concentrations ( Thorpe et al. , 2005 ; Van Bel and Hafke, 2005 ). These variables may manipulate the proton-motive force, which drives the sucrose carriers. K + and Ca 2+ channels have been found on the plasma membrane of the SECCCs in the transport phloem ( Deeken et al. , 2000 , 2002 ; Van Bel and Hafke, 2005 ).
It has been speculated that, in addition to short-term buffering, the leakage-retrieval mechanism also plays a role in maintaining the pressure gradient along the phloem pathway ( van Bel, 2003 b , 2005 ). It is believed that the transport corridors in the sieve plates are so narrow that appreciable losses in turgor pressure arise at every sieve plate. Therefore, van Bel (2003 b ; van Bel and Hafke, 2005 ) hypothesizes that solutes are released in the sieve tube apoplast at the proximal side of the sieve plate and that they are retrieved at the distal side ( Fig. 2 ). Membrane channels should facilitate these leakage and retrieval processes around the sieve plates. In this view, the leakage-retrieval system would lead to a re-establishment of the turgor pressure behind every sieve plate. This proposed mechanism is a variation on the original relay hypothesis, as van Bel (2003 b ; van Bel and Hafke, 2005 ) does not state that sieve tubes are shorter than the plant’s axis. In their concept, relays are present at the sieve plates of the sieve tube, while in the original relay hypothesis relays are only present where individual ‘shorter’ sieve tubes are in vertical contact.

The pressure drop around the sieve tubes is reduced if the leakage-retrieval mechanism acts as a dynamic relay system. (A) Pressure drop over the sieve plates due to the plates’ higher resistance towards sucrose transport. (B) A reduced pressure drop due to sucrose leakage proximal of sieve plates and retrieval at the distal site.
Furthermore, it is suggested that the flow between the long-term lateral sinks and the SECCCs is controlled by their apoplastic carbohydrate concentration ( McQueen et al. , 2005 ; Thorpe et al. , 2005 ). These long-term sinks relate to long-term buffering of stored carbohydrate reserves in the symplast of parenchyma tissues and stem ray cells. The proposed hypothesis for long-term buffering assumes that the short-term apoplastic phloem concentration is rather constant ( van Bel, 1990 ). Whenever the carbohydrate concentration in the apoplast is either substantially increased or decreased by short-term buffering, the long-term symplastic pool will be replenished or depleted, respectively ( Fig. 3 ). According to this hypothesis, the apoplastic sugar concentration controls the replenishment and remobilization of the long-term lateral stem storage tissues. The short-term buffering capacity depends on the volume of the apoplastic pool and its apoplastic sugar concentration, while the long-term buffering depends on the amount of available storage tissues and their carbon concentration. When the limits of long-term lateral buffering are reached, the activity of the sources and sinks needs to be regulated. The storage capability is probably adapted to predictable imbalances of supply and demand, such as in diurnal and seasonal trends, or it could develop as the need arises ( Thorpe et al. , 2005 ).

Schematic presentation of the pathways for lateral transport of carbohydrates in the plant stem. (1) From the phloem sieve tubes (Pc), carbohydrates can move by diffusion via plasmodesmata into the symplastic storage compartment (S) (e.g. ray cells or phloem parenchyma). Carbohydrates can diffuse through the cell membrane (2) into the apoplast (A) or (3) into the storage compartment. From the apoplast adjacent to the phloem, carbohydrates can be (4) actively retrieved (reloaded) into phloem, (5) be loaded into the storage compartment, or (6) diffuse further through the apoplast into the xylem stream (X), where flow is usually in the opposite direction of that in the phloem (Reprinted from Vascular transport in plants . Thorpe MR, Minchin PEH, Gould N, McQueen JC. 2005. The stem apoplast: a potential communication channel in plant growth regulation. In: Holbrook NM, Zwieniecki MA, eds. Burlington: Elsevier, 355–371, with permission from Elsevier).
Phloem functioning is not limited to translocation of sugars between sources and sinks, but is also involved in long-distance signalling and plant defence.
Long-distance signalling relates to the distribution of a local effect, mostly induced by changes in source and sink activity, over long distances. The first way to conduct this physicochemical information transfer between sources and sinks is in the form of pressure–concentration waves ( Thompson and Holbrook, 2003 b , 2004 ; Thompson, 2006 ). A theoretical study ( Thompson and Holbrook, 2003 b ) has shown that these pressure–concentration waves will travel faster than the phloem sap itself, but only if the osmotic pressure is high relative to the turgor difference between sources and sinks ( Thompson, 2006 ). This first assumption is one of the reasons why Thompson and Holbrook (2003 b , 2004 ; Thompson, 2006 ) are convinced that the source–sink pressure gradient is small and the osmotic potential of the phloem tubes is high. Furthermore, Thompson and Holbrook (2003 b , 2004 ; Thompson, 2006 ) assume that the total water potential of the apoplast and the symplast of the SECCC are in equilibrium along the phloem pathway. This second assumption implicates that every change in turgor is accompanied by a proportional change in solute concentration. For example, when a turgor drop is induced, the total water potential of the phloem sap will become lower (more negative) compared with that of the surrounding apoplast. As such, the sieve tube is forced to absorb more water from the apoplast and consequentially the phloem sap is diluted ( Thompson, 2006 ). If these two assumptions as previously mentioned are fulfilled, pressure–concentration waves can explain long-distance signalling as depicted in Fig. 4 . A local increase in solute concentration leads to an influx of water and, hence, an increase in local turgor ( Fig. 4A , B ). This turgor disturbance can be propagated as a pressure–concentration wave along the length of the sieve tube. The water potential of the sap transiently increases (becomes less negative) due to the increased turgor ( Fig. 4C ), resulting in an efflux of water that concentrates the already present solutes until equilibrium in water potential is regained ( Fig. 4D ) ( Thompson and Holbrook, 2004 ). This example illustrates how the pressure–concentration waves can alter the turgor pressure in the entire sieve tube in response to a local induced disturbance. Therefore, unloading and loading rates along the entire phloem pathway (i.e. source, transport, and sink phloem) will alter correspondingly to the changed turgor pressure, because the (un)loading processes are controlled by the SECCC turgor pressure as previously discussed. This concept is physiologically very interesting because individual cells in the decentralized plant body can only sense and modify their own turgor ( Thompson, 2006 ). If relays are present along the transport phloem, it seems logical that the wave propagation will be stopped at each relay as this physically interrupts the phloem symplast. In the case of the dynamic relay hypothesis based on the leakage-retrieval mechanism, the pressure wave will probably not be halted at the sieve plates as the phloem symplast is not interrupted.

The propagation of pressure–concentration waves. At steady state, solute (S) and apoplastic water flux (f) into the sieve tube equal the solute and water flux out. The size of these symbols and their associated arrows denote the magnitude of each flux. The concentration of open circles is proportional to the sugar concentration, and the degree of shading in the background is proportional to turgor pressure. It is assumed that in the sieve tube the osmotic potential is high relative to the source–sink pressure gradient and that no external gradient occurs in the apoplastic water potential. (A) The pressure and solute concentration are initially low. (B) Solute loading in the collection phloem increases, which locally increases concentration, membrane water influx, and pressure. (C) This increase in pressure is rapidly propagated along the length of the sieve tube, transiently raising the sap water potential relative to the apoplast and the efflux of water near the release phloem. (D) The terminal efflux of water concentrates the solutes, raising the solute concentration until the sieve sap is in local water potential equilibrium (from Thompson and Holbrook, 2004 . Scaling phloem transport: information transmission. Plant, Cell and Environment 27, 509–519 with permission © John Wiley & Sons Ltd).
Long-distance signalling by the phloem can also be achieved through transport of hormones ( Turgeon and Wolf, 2009 ) and macromolecules, such as RNA and proteins ( Oparka and Cruz, 2000 ; van Bel, 2003 b ; Thorpe et al. , 2005 ; Pritchard, 2007 ; Turgeon and Wolf, 2009 ). It is well accepted that low molecular weight signalling molecules are translocated in the phloem, initiating physiologically amplified responses at a distance from their sites of synthesis ( Thompson and Schulz, 1999 ). Several investigators ( Baker, 2000 ; Friml and Palme, 2002 ; Turgeon and Wolf, 2009 ) have detected the presence of the endogenous plant hormone auxin and its native form, indole-3-acetic acid (IAA), in the phloem sap. Peptide hormones, such as systemin, also appear to be transported throughout the plant via the phloem ( Narvaez-Vasquez et al. , 1995 ; Thompson and Schulz, 1999 ). Some mRNA molecules present in the phloem sap are transported with the phloem sap towards the sinks where they seem to induce gene silencing ( Oparka and Cruz, 2000 ; van Bel, 2003 b ). However, the generality of the concept that mRNA molecules act as signals to coordinate developmental processes at the whole-plant level is still under debate ( Turgeon and Wolf, 2009 ). Long-distance trafficking of some protein products in the phloem is associated with the promotion of flowering in several species ( Corbesier et al. , 2007 ; Lin et al. , 2007 ; Tamaki et al. , 2007 ; Turgeon and Wolf, 2009 ). Notwithstanding that some phloem proteins are involved in long-distance signalling, it is believed that many of the phloem proteins have entered the sieve element by non-specific diffusion and not by design ( Oparka and Cruz, 2000 ; Turgeon and Wolf, 2009 ).
Finally, profound and sudden physiological changes can be communicated over large distances by electropotential waves ( Furch et al. , 2010 ). These waves have been recorded in response to stimuli such as wounding, cold, heat, and electrical shocks ( Fromm and Spanswick, 1993 ; Rhodes et al. , 1996 ; Mancuso, 1999 ; Furch et al. , 2010 ). Along their path, electropotential waves often cause occlusion of the sieve elements by callose and sealing proteins ( Furch et al. , 2010 ).
Besides long-distance signalling, phloem plays a role in plant defence against predators. First, the phloem protects the plant by its high turgor pressure and concomitant high sugar concentration in the SECCCs ( Turgeon, 2010 a ). When a sieve tube is wounded, pressure release causes surging and forces cellular debris into the sieve pores, sealing the phloem (e.g. Ehlers et al. , 2000 ). Some insects have adapted and can maintain their feeding due to specific compounds in their saliva ( Will and van Bel, 2006 ), but the majority of them cannot cope with this effective sealing strategy ( Turgeon, 2010 a ). In addition, the high osmotic pressure of the phloem discourages the phloem feeders as it desiccates the animal tissues ( Turgeon, 2010 a ). Secondly, the plant is protected from predators by phloem proteins and secondary compounds in the phloem. Specific non-dispersive phloem proteins, for example P-proteins or forisomes, are able to seal the sieve elements at the sieve plate after damage so that leaking of the pressurized phloem sap out of the damage sieve tube is prevented ( van Bel et al. , 2002 ; Knoblauch and Peters, 2010 ; Ernst et al. , 2012 ). Several phloem proteins, for example serpin ( Petersen et al. , 2005 ) and systemin ( Narvaez-Vasquez et al. , 1995 ), inhibit the protein digestion of insects and hence protect against chewing insects ( van Bel, 2003 b ; Pritchard, 2007 ). In addition, the phloem transports several types of secondary compounds, which are toxic and behave as natural pesticides. Examples of such secondary compounds are glucosinolates, iridoid glycosides, pyrrolizidine alkaloids, and cardenolides ( Turgeon and Wolf, 2009 ).
The Münch theory of phloem transport is continuously being refined by including both active and passive phloem (un)loading in the collection and release phloem and by introducing the leakage-retrieval process along the transport phloem. Phloem-related data measured on trees and herbaceous species revealed significant differences between both plant types. Not only do their loading strategies differ, but also the observed phloem pressure is much higher in herbs than in trees, although Münch’s theory suggested the opposite. Based on these findings the abandoned hypothesis of a relay system needs to be reassessed, especially for trees. Therefore, we urge that future experiments focus not only on herbs (which is mostly the case in current studies), but also on trees. Most of the phloem hypotheses (e.g. small phloem pressure gradient, leakage-retrieval process, relay system) are based on fragmentary observed data or theoretical experiments. Nevertheless, they are experimentally hard to test due to the technically demanding measurement methods associated with the protective nature of phloem tissue. In particular, information about phloem anatomy (sieve tube length, sieve pore areas) and phloem pressure seems crucial to elucidate the proposed hypotheses. A better fundamental understanding of the phloem system could increase our insight into how information is exchanged between sources and sinks and how growth is finally regulated. Hence, it could be a valuable tool to increase the productivity of and mitigate disastrous environmental impacts on commercial plants and natural forests.
We thank the Special Research Fund (B.O.F.) of Ghent University for the post-doc funding granted to VDS.
Baker D . 2000 . Vascular transport of auxins and cytokinins in Ricinus . Plant Growth Regulation 32 , 157 – 160 .
Google Scholar
Barker L Kuhn C Weise A Schulz A Gebhardt C Hirner B Hellmann H Schulze W Ward JM Frommer WB . 2000 . SUT2, a putative sucrose sensor in sieve elements . The Plant Cell 12 , 1153 – 1164 .
Cheng YH Arakawa O Kasai M Sawada S . 2008 . Analysis of reduced photosynthesis in the apple leaf under sink-limited conditions due to girdling . Journal of the Japanese Society for Horticultural Science 77 , 115 – 121 .
Corbesier L Vincent C Jang S et al. 2007 . FT protein movement contributes to long-distance signaling in floral induction of Arabidopsis . Science 316 , 1030 – 1033 .
Daie J . 1989 . Turgor-regulated sugar release from the source leaves of sugarbeet ( Beta vulgaris L) . Plant and Cell Physiology 30 , 1115 – 1121 .
Dannoura M Maillard P Fresneau C et al. 2011 . In situ assessment of the velocity of carbon transfer by tracing 13C in trunk CO2 efflux after pulse labelling: variations among tree species and seasons . New Phytologist 190 , 181 – 192 .
De Schepper V Bühler J Thorpe M Roeb G Huber G van Dusschoten D Jahnke J Steppe K . 2013 . 11C-PET imaging reveals transport dynamics and sectorial plasticity of oak phloem after girdling . Frontiers in Plant Science 4 , 200 .
Deeken R Geiger D Fromm J Koroleva O Ache P Langenfeld-Heyser R Sauer N May ST Hedrich R . 2002 . Loss of the AKT2/3 potassium channel affects sugar loading into the phloem of Arabidopsis . Planta 216 , 334 – 344 .
Deeken R Sanders C Ache P Hedrich R . 2000 . Developmental and light-dependent regulation of a phloem-localised K+ channel of Arabidopsis thaliana . The Plant Journal 23 , 285 – 290 .
Ehlers K Knoblauch M van Bel AJE . 2000 . Ultrastructural features of well-preserved and injured sieve elements: minute clamps keep the phloem transport conduits free for mass flow . Protoplasma 214 , 80 – 92 .
Ernst AM Jekat SB Zielonka S Muller B Neumann U Ruping B Twyman RM Krzyzanek V Prufer D Noll GA . 2012 . Sieve element occlusion (SEO) genes encode structural phloem proteins involved in wound sealing of the phloem . Proceedings of the National Academy of Sciences, USA 109 , E1980 – E1989 .
Fisher DB . 1978 . Evaluation of Münch hypothesis for phloem transport in soybean . Planta 139 , 25 – 28 .
Fisher DB Cash-Clark CE . 2000 . Sieve tube unloading and post-phloem transport of fluorescent tracers and proteins injected into sieve tubes via severed aphid stylets . Plant Physiology 123 , 125 – 137 .
Foyer CH . 1988 . Feedback inhibition of photosynthesis through source–sink regulation in leaves . Plant Physiology and Biochemistry 26 , 483 – 492 .
Friml J Palme K . 2002 . Polar auxin transport—old questions and new concepts? Plant Molecular Biology 49 , 273 – 284 .
Fromm J Spanswick R . 1993 . Characteristics of action-potentials in willow ( Salix viminalis L.) . Journal of Experimental Botany 44 , 1119 – 1125 .
Furch ACU Zimmermann MR Will T Hafke JB van Bel AJE . 2010 . Remote-controlled stop of phloem mass flow by biphasic occlusion in Cucurbita maxima . Journal of Experimental Botany 61 , 3697 – 3708 .
Gamalei YV . 2002 . Assimilate transport and partitioning in plants: approaches, methods, and facets of research . Russian Journal of Plant Physiology 49 , 16 – 31 .
Gould N Minchin PEH Thorpe MR . 2004 . Direct measurements of sieve element hydrostatic pressure reveal strong regulation after pathway blockage . Functional Plant Biology 31 , 987 – 993 .
Gould N Thorpe MR Koroleva O Minchin PEH . 2005 . Phloem hydrostatic pressure relates to solute loading rate: a direct test of the Munch hypothesis . Functional Plant Biology 32 , 1019 – 1026 .
Hammel HT . 1968 . Measurement of turgor pressure and its gradient in phloem of oak . Plant Physiology 43 , 1042 – 1048 .
Harper JL . 1989 . The value of a leaf . Oecologia 80 , 53 – 58 .
Hölttä T Mencuccini M Nikinmaa E . 2009 . Linking phloem function to structure: analysis with a coupled xylem–phloem transport model . Journal of Theoretical Biology 259 , 325 – 337 .
Jahnke S Schlesinger U Feige GB Knust EJ . 1998 . Transport of photoassimilates in young trees of Fraxinus and Sorbus: measurement of translocation in vivo . Botanica Acta 111 , 307 – 315 .
Jensen KH Lee J Bohr T Bruus H Holbrook NM Zwieniecki MA . 2011 . Optimality of the Munch mechanism for translocation of sugars in plants . Journal of the Royal Society Interface 8 , 1155 – 1165 .
Jensen KH Liesche J Bohr T Schulz A . 2012 . Universality of phloem transport in seed plants . Plant, Cell and Environment 35 , 1065 – 1076 .
Jensen KH Rio E Hansen R Clanet C Bohr T . 2009 . Osmotically driven pipe flows and their relation to sugar transport in plants . Journal of Fluid Mechanics 636 , 371 – 396 .
Knoblauch M Peters WS . 2010 . Munch, morphology, microfluidics—our structural problem with the phloem . Plant, Cell and Environment 33 , 1439 – 1452 .
Köckenberger W Pope JM Xia Y Jeffrey KR Komor E Callaghan PT . 1997 . A non-invasive measurement of phloem and xylem water flow in castor bean seedlings by nuclear magnetic resonance microimaging . Planta 201 , 53 – 63 .
Komor E . 2000 . Source physiology and assimilate transport: the interaction of sucrose metabolism, starch storage and phloem export in source leaves and the effects on sugar status in phloem . Australian Journal of Plant Physiology 27 , 497 – 505 .
Kuhn AJ Schröder WH Bauch J . 1997 . On the distribution and transport of mineral elements in xylem, cambium and phloem of spruce ( Picea abies [L.] Karst.) . Holzforschung 51 , 487 – 496 .
Lalonde S Boles E Hellmann H Barker L Patrick JW Frommer WB Ward JM . 1999 . The dual function of sugar carriers: transport and sugar sensing . The Plant Cell 11 , 707 – 726 .
Lalonde S Tegeder M Throne-Holst M Frommer WB Patrick JW . 2003 . Phloem loading and unloading of sugars and amino acids . Plant, Cell and Environment 26 , 37 – 56 .
Lambers H Chapin FS Pons TL . 1998 . Plant physiological ecology . New York : Springer-Verlag .
Google Preview
Lang A . 1979 . Relay mechanism for phloem translocation . Annals of Botany 44 , 141 – 145 .
Lewis CE Noctor G Causton D Foyer CH . 2000 . Regulation of assimilate partitioning in leaves . Australian Journal of Plant Physiology 27 , 507 – 519 .
Liesche J Martens HJ Schulz A . 2011 . Symplasmic transport and phloem loading in gymnosperm leaves . Protoplasma 248 , 181 – 190 .
Liesche J Schulz A Jensen KH Minchin P Bohr T . 2013 . Theoretical and experimental determination of phloem translocation speeds in gymnosperm and angiosperm trees . In: Steppe K , ed. Ninth international workshop on sap flow , Vol. 991 . Ghent : ISHS .
Lin MK Belanger H Lee YJ et al. 2007 . FLOWERING LOCUS T protein may act as the long-distance florigenic signal in the cucurbits . The Plant Cell 19 , 1488 – 1506 .
Mancuso S . 1999 . Hydraulic and electrical transmission of wound-induced signals in Vitis vinifera . Australian Journal of Plant Physiology 26 , 55 – 61 .
McQueen JC Minchin PEH Thorpe MR Silvester WB . 2005 . Short-term storage of carbohydrate in stem tissue of apple ( Malus domestica ), a woody perennial: evidence for involvement of the apoplast . Functional Plant Biology 32 , 1027 – 1031 .
Minchin PEH Thorpe MR . 1987 . Measurement of unloading and reloading of photo-assimilates within the stem of bean . Journal of Experimental Botany 38 , 211 – 220 .
Minchin PEH Thorpe MR Farrar JF Koroleva OA . 2002 . Source–sink coupling in young barley plants and control of phloem loading . Journal of Experimental Botany 53 , 1671 – 1676 .
Mullendore DL Windt CW Van As H Knoblauch M . 2010 . Sieve tube geometry in relation to phloem flow . The Plant Cell 22 , 579 – 593 .
Münch E . 1930 . Die Stoffbewegunen in der Pflanze . Jena : Verlag von Gustav Fischer .
Murphy R Aikman DP . 1989 . An investigation of the relay hypothesis of phloem transport in Ricinus communis L . Journal of Experimental Botany 40 , 1079 – 1088 .
Narvaez-Vasquez J Pearce G Orozco-Cardenas ML Franceschi VR Ryan CA . 1995 . Autoradiographic and biochemical evidence for the systemic translocation of sytemin in tomato plants . Planta 195 , 593 – 600 .
Oparka KJ Cruz SS . 2000 . The great escape: phloem transport and unloading of macromolecules . Annual Review of Plant Physiology and Plant Molecular Biology 51 , 323 – 347 .
Orlich G Hofbruckl M Schulz A . 1998 . A symplasmic flow of sucrose contributes to phloem loading in Ricinus cotyledons . Planta 206 , 108 – 116 .
Patrick JW . 1990 . Sieve element unloading—cellular pathway, mechanism and control . Physiologia Plantarum 78 , 298 – 308 .
Patrick JW Offler CE . 1996 . Post-sieve element transport of photoassimilates in sink regions . Journal of Experimental Botany 47 , 1165 – 1177 .
Patrick JW Zhang WH Tyerman SD Offler CE Walker NA . 2001 . Role of membrane transport in phloem translocation of assimilates and water . Australian Journal of Plant Physiology 28 , 695 – 707 .
Petersen ML Hejgaard J Thompson GA Schulz A . 2005 . Cucurbit phloem serpins are graft-transmissible and appear to be resistant to turnover in the sieve element–companion cell complex . Journal of Experimental Botany 56 , 3111 – 3120 .
Pittermann J . 2010 . The evolution of water transport in plants: an integrated approach . Geobiology 8 , 112 – 139 .
Pritchard J . 2007 . Solute transport in the phloem . In: Yeo AR Flowers TJ , eds. Plant solute transport . Oxford : Blackwell Publishing , 235 – 274 .
Rennie EA Turgeon R . 2009 . A comprehensive picture of phloem loading strategies . Proceedings of the National Academy of Sciences, USA 106 , 14162 – 14167 .
Rhodes JD Thain JF Wildon DC . 1996 . The pathway for systemic electrical signal conduction in the wounded tomato plant . Planta 200 , 50 – 57 .
Rosner S Baier P Kikuta SB . 2001 . Osmotic potential of Norway spruce [ Picea abies (L.) Karst.] secondary phloem in relation to anatomy . Trees-Structure and Function 15 , 472 – 482 .
Sovonick-Dunford S Lee DR Zimmermann MH . 1981 . Direct and indirect measurements of phloem turgor pressure in White Ash . Plant Physiology 68 , 121 – 126 .
Tamaki S Matsuo S Wong HL Yokoi S Shimamoto K . 2007 . Hd3a protein is a mobile flowering signal in rice . Science 316 , 1033 – 1036 .
Thompson GA Schulz A . 1999 . Macromolecular trafficking in the phloem . Trends in Plant Science 4 , 354 – 360 .
Thompson MV . 2006 . Phloem: the long and the short of it . Trends in Plant Science 11 , 26 – 32 .
Thompson MV Holbrook NM . 2003a . Application of a single-solute non-steady-state phloem model to the study of long-distance assimilate transport . Journal of Theoretical Biology 220 , 419 – 455 .
Thompson MV Holbrook NM . 2003b . Scaling phloem transport: water potential equilibrium and osmoregulatory flow . Plant, Cell and Environment 26 , 1561 – 1577 .
Thompson MV Holbrook NM . 2004 . Scaling phloem transport: information transmission . Plant, Cell and Environment 27 , 509 – 519 .
Thorpe MR Lang A . 1983 . Control of import and export of photosynthate in leaves . Journal of Experimental Botany 34 , 231 – 239 .
Thorpe MR Minchin PEH Gould N McQueen JC . 2005 . The stem apoplast: a potential communication channel in plant growth regulation . In: Holbrook NM Zwieniecki MA , eds. Vascular transport in plants . Burlington : Elsevier , 355 – 371 .
Turgeon R . 2010a . The puzzle of phloem pressure . Plant Physiology 154 , 578 – 581 .
Turgeon R . 2010b . The role of phloem loading reconsidered . Plant Physiology 152 , 1817 – 1823 .
Turgeon R Ayre BG . 2005 . Pathways and mechanism of phloem loading . In: Holbrook NM Zwieniecki MA , eds. Vascular transport in plants . Burlington : Elsevier , 45 – 67 .
Turgeon R Wolf S . 2009 . Phloem transport: cellular pathways and molecular trafficking . Annual Review of Plant Biology 60 , 207 – 221 .
van Bel AJE . 1990 . Xylem–phloem exchange via the rays—the undevalued route of transport . Journal of Experimental Botany 41 , 631 – 644 .
van Bel AJE . 1996 . Interaction between sieve element and companion cell and the consequences for photoassimilate distribution. Two structural hardware frames with associated physiological software packages in dicotyledons . Journal of Experimental Botany 47 , 1129 – 1140 .
van Bel AJE . 2003a . Phloem transport: the collective power of single modules . In: Larcher W , ed. Physiological plant ecology . New York : Springer-Verlag , 151 – 155 .
van Bel AJE . 2003b . The phloem, a miracle of ingenuity . Plant, Cell and Environment 26 , 125 – 149 .
van Bel AJE . 2003c . Transport phloem: low profile, high impact . Plant Physiology 131 , 1509 – 1510 .
van Bel AJE Ehlers K Knoblauch M . 2002 . Sieve elements caught in the act . Trends in Plant Science 7 , 126 – 132 .
Van Bel AJE Hafke JB . 2005 . Physiological determinants of phloem transport . In: Holbrook NM Zwieniecki MA , eds. Vascular transport in plants . Burlington : Elsevier , 19 – 44 .
Vaughn MW Harrington GN Bush DR . 2002 . Sucrose-mediated transcriptional regulation of sucrose symporter activity in the phloem . Proceedings of the National Academy of Sciences, USA 99 , 10876 – 10880 .
Will T van Bel AJE . 2006 . Physical and chemical interactions between aphids and plants . Journal of Experimental Botany 57 , 729 – 737 .
Willenbrink J . 2002 . Assimilate transport in phloem: regulation and mechanism . Russian Journal of Plant Physiology 49 , 8 – 15 .
Windt CW Vergeldt FJ De Jager PA Van As H . 2006 . MRI of long-distance water transport: a comparison of the phloem and xylem flow characteristics and dynamics in poplar, castor bean, tomato and tobacco . Plant, Cell and Environment 29 , 1715 – 1729 .
Wright JP Fisher DB . 1980 . Direct measurement of sieve tube turgor pressure using severed aphid stylets . Plant Physiology 65 , 1133 – 1135 .
Email alerts
Citing articles via.
- Recommend to your Library
Affiliations
- Online ISSN 1460-2431
- Print ISSN 0022-0957
- Copyright © 2024 Society for Experimental Biology
- About Oxford Academic
- Publish journals with us
- University press partners
- What we publish
- New features
- Open access
- Institutional account management
- Rights and permissions
- Get help with access
- Accessibility
- Advertising
- Media enquiries
- Oxford University Press
- Oxford Languages
- University of Oxford
Oxford University Press is a department of the University of Oxford. It furthers the University's objective of excellence in research, scholarship, and education by publishing worldwide
- Copyright © 2024 Oxford University Press
- Cookie settings
- Cookie policy
- Privacy policy
- Legal notice
This Feature Is Available To Subscribers Only
Sign In or Create an Account
This PDF is available to Subscribers Only
For full access to this pdf, sign in to an existing account, or purchase an annual subscription.
- Biology Article
Mass Flow Hypothesis
Table of Contents
Introduction
The Mass Flow Hypothesis was first proposed by German plant physiologist Ernst Munch in the year 1930. He theorised the movement of sap through the phloem tissue in plants. This theory is also known as the Pressure Flow Hypothesis. A highly concentrated organic sugar especially sugar in the cells of the phloem from a source like a leaf forms a diffusion gradient which draws water into the cells of phloem tissue from the adjacent xylem. This develops turgor pressure in the phloem, which is also called hydrostatic pressure.
Phloem movement occurs by mass flow from sources of sugar to sugar sinks. The phloem movement is bidirectional but unidirectional in xylem cells. Due to this multidirectional flow, it is not uncommon for sap in the sieve tubes besides to move in opposite directions based on the fact that sap cannot travel easily between adjacent sieve tubes.
When the movement of minerals and water via the xylem is driven mostly by negative pressure and movement via phloem is driven by hydrostatic pressure. This process is called translocation and is accompanied by a process known as phloem loading and unloading. Cells in sugar sources load a sieve tube by osmosis developing pressure that pushes the sap low. The cells deliver solutes out of the elements of sieve tube and produce opposite effects. The sugar gradient from the source creates pressure-flow via the sieve tube towards the sink.
- Glucose is formed by photosynthesis in the cells of mesophyll and some glucose is utilized in the cells during respiration. The leftover glucose is transformed into non-reducing sugar.
- Sucrose is delivered to the neighbour cells of minute veins of the leaves.
- Sucrose diffuses from neighbour cells to the elements of the sieve tube via plasmodesmata. Hence, the amount of sucrose rises in the elements of the sieve tube.
- Water travels from the close xylem to the leaf vein by osmosis and raises the hydrostatic pressure of the elements of the sieve tube.
- The Hydrostatic pressure shifts the sucrose along with other substances via the cell of the sieve tube towards the sink.
- In storage sinks, sucrose is eliminated into the apoplast before entering the sink’s symplast.
- The water travels out of the cells via osmosis and lowers the hydrostatic pressure in them. Hence, a gradient of pressure is developed as a result of the entry of sugar at the source and elimination of sucrose at the sink.
- The phloem sugar is eradicated by the cortex of the root and stem and utilized by cellular respiration. The starch is insoluble and does not exert any osmotic effect. Ultimately, pure water is left and drawn into xylem vessels by transpiration pull.
To know more about mass flow hypothesis, visit BYJU’S.
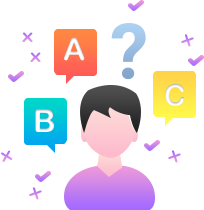
Put your understanding of this concept to test by answering a few MCQs. Click ‘Start Quiz’ to begin!
Select the correct answer and click on the “Finish” button Check your score and answers at the end of the quiz
Visit BYJU’S for all Biology related queries and study materials
Your result is as below
Request OTP on Voice Call
Leave a Comment Cancel reply
Your Mobile number and Email id will not be published. Required fields are marked *
Post My Comment

what observations were not explained in the Munch’s mass flow

- Share Share
Register with BYJU'S & Download Free PDFs
Register with byju's & watch live videos.

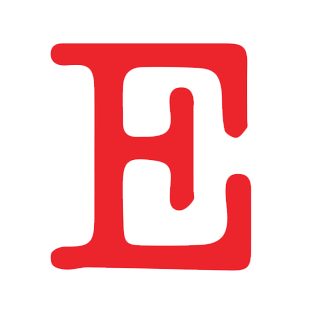
- Scholarly Community Encyclopedia
- Log in/Sign up

Video Upload Options
- MDPI and ACS Style
- Chicago Style
The Pressure Flow Hypothesis, also known as the Mass Flow Hypothesis, is the best-supported theory to explain the movement of sap through the phloem. It was proposed by Ernst Munch, a Germany plant physiologist in 1930. A high concentration of organic substances, particularly sugar, inside cells of the phloem at a source, such as a leaf, creates a diffusion gradient (osmotic gradient) that draws water into the cells from the adjacent xylem. This creates turgor pressure, also known as hydrostatic pressure, in the phloem. Movement of phloem sap occurs by bulk flow (mass flow) from sugar sources to sugar sinks. The movement in phloem is bidirectional, whereas, in xylem cells, it is unidirectional (upward). Because of this multi-directional flow, coupled with the fact that sap cannot move with ease between adjacent sieve-tubes, it is not unusual for sap in adjacent sieve-tubes to be flowing in opposite directions.
1. Sources and Sinks
A sugar source is any part of the plant that is producing or releasing sugar.
During the plant's growth period, usually during the spring, storage organs such as the roots are sugar sources, and the plant's many growing areas are sugar sinks.
After the growth period, when the meristems are dormant, the leaves are sources, and storage organs are sinks. Developing seed-bearing organs (such as fruit) are always sinks.
2. Mechanisms
While movement of water and minerals through the xylem is driven by negative pressures (tension) most of the time, movement through the phloem is driven by positive hydrostatic pressure. This process is termed translocation , and is accomplished by a process called phloem loading and unloading . Cells in a sugar source "load" a sieve-tube element by actively transporting solute molecules into it. This causes water to move into the sieve-tube element by osmosis, creating pressure that pushes the sap down the tube. In sugar sinks, cells actively transport solutes out of the sieve-tube elements, producing the exactly opposite effect. The gradient of sugar from source to sink causes pressure flow through the sieve tube toward the sink.
The mechanisms are as follows:
- Glucose is produced by photosynthesis in the mesophyll cells of green leaves. Some glucose is used within the cells during respiration. The rest of the glucose is converted into non-reducing sugar i.e. sucrose. It has been shown that the sucrose concentration in sieve tubes in leaves is commonly between 10 and 30 percent whereas it forms only 0.5% solution in the photosynthesis cells.
- The sucrose is actively transported to the companion cells of the smallest veins in the leaves.
- The sucrose diffuses through the plasmodesmata from the companion cells to the sieve tube elements. As a result, concentration of sucrose increases in the sieve tube elements.
- Water moves by osmosis from the nearby xylem in the same leaf vein. This increases the hydrostatic pressure of the sieve tube elements.
- Hydrostatic pressure moves the sucrose and other substances through the sieve tube cells, towards a sink.
- In the storage sinks, such as sugar beet root and sugar cane stem, sucrose is removed into apoplast prior to entering the symplast of the sink.
- Water moves out of the sieve tube cells by osmosis, lowering the hydrostatic pressure within them. Thus the pressure gradient is established as a consequence of entry of sugars in sieve elements at the source and removal of sucrose at the sink. The presence of sieve plates greatly increases the resistance along the pathway and results in the generation and maintenance of substantial pressure gradients in the sieve elements between source and sink.
- The phloem sugar is removed by the cortex of both stem and root, and is consumed by cellular respiration or else converted into starch. Starch is insoluble and exerts no osmotic effect. Consequently, the osmotic pressure of the contents of phloem decreases. Finally relatively pure water is left in the phloem and this is thought to leave by osmosis or be drawn back into nearby xylem vessels by suction of the transpiration pull.
The pressure flow mechanism depends upon:
- Turgor pressure
- Difference of osmotic pressure gradient along the direction of flow between the source and the sink.

3. Evidence
There are different pieces of evidences that support the hypothesis. Firstly, there is an exudation of solution from the phloem when the stem is cut or punctured by the mouthparts of an aphid, a classical experiment demonstrating the translocation function of phloem, indicating that the phloem sap is under pressure. Secondly, concentration gradients of organic solutes are proved to be present between the sink and the source. Thirdly, when viruses or growth chemicals are applied to a well-illuminated (actively photosynthesising) leaf, they are translocated downwards to the roots. Yet, when applied to shaded leaves, such downward translocation of chemicals does not occur, hence showing that diffusion is not a possible process involved in translocation.
4. Criticisms
Opposition or criticisms against the hypothesis are often voiced. Some argue that mass flow is a passive process while sieve tube vessels are supported by companion cells. Hence, the hypothesis neglects the living nature of phloem. Moreover, it is found that amino acids and sugars (examples of organic solutes) are translocated at different rates, which is contrary to the assumption in the hypothesis that all materials being transported would travel at uniform speed. Bi-directional movements of solutes in translocation process as well as the fact that translocation is heavily affected by changes in environmental conditions like temperature and metabolic inhibitors are two defects of the hypothesis.
An objection leveled against the pressure flow mechanism is that it does not explain the phenomenon of bidirectional movement i.e. movement of different substances in opponent directions at the same time. The phenomenon of bidirectional movement can be demonstrated by applying two different substances at the same time to the phloem of a stem at two different points, and following their longitudinal movement along the stem. If the mechanism of translocation operates according to pressure flow hypothesis, bidirectional movement in a single sieve tube is not possible. Experiments to demonstrate bidirectional movement in a single sieve tube are technically very difficult to perform. Some experiments indicate that bidirectional movement may occur in a single sieve tube, whereas others do not.
5. Other Theories
Some plants appear not to load phloem by active transport. In these cases a mechanism known as the polymer trap mechanism was proposed by Robert Turgeon. [ 1 ] In this case small sugars such as sucrose move into intermediary cells through narrow plasmodesmata, where they are polymerised to raffinose and other larger oligosaccharides. Now they are unable to move back, but can proceed through wider plasmodesmata into the sieve tube element.
The symplastic phloem loading is confined mostly to plants in tropical rain forests and is seen as more primitive. The actively transported apoplastic phloem loading is viewed as more advanced, as it is found in the later-evolved plants, and particularly in those in temperate and arid conditions. This mechanism may therefore have allowed plants to colonise the cooler locations.
Organic molecules such as sugars, amino acids, certain hormones, and even messenger RNAs are transported in the phloem through sieve tube elements.
- Turgeon, R (1991). "Symplastic phloem loading and the sink-source transition in leaves: a model". Recent Advances Phloem Transport and Assimilate Compartmentation.
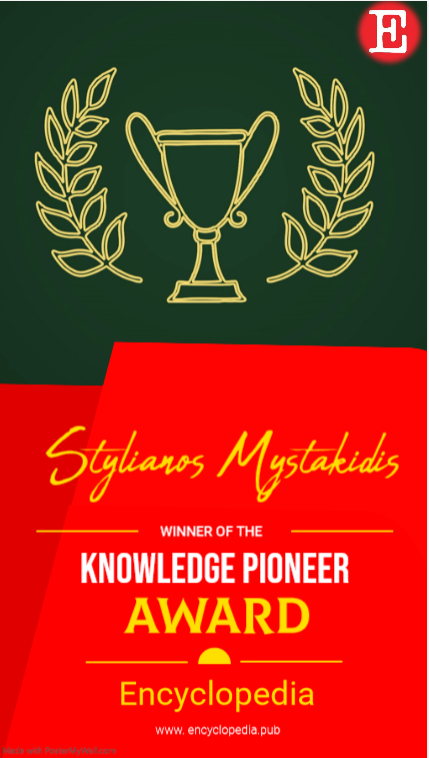
- Terms and Conditions
- Privacy Policy
- Advisory Board


An official website of the United States government
The .gov means it’s official. Federal government websites often end in .gov or .mil. Before sharing sensitive information, make sure you’re on a federal government site.
The site is secure. The https:// ensures that you are connecting to the official website and that any information you provide is encrypted and transmitted securely.
- Publications
- Account settings
Preview improvements coming to the PMC website in October 2024. Learn More or Try it out now .
- Advanced Search
- Journal List
- Plant Physiol
- v.154(2); 2010 Oct

The Puzzle of Phloem Pressure 1
Although transport of organic nutrients and regulatory molecules in the phloem underlies all aspects of growth and development, we still cannot say with confidence that we adequately understand how it is motivated. A major problem is that it is difficult to reconcile the theory of pressure flow, proposed over 80 years ago by Ernst Münch (1927) , with transport in large trees. Significantly, phloem pressure does not scale to plant size. Also, the very high turgor in the phloem of herbs suggests that pressure has functions beyond that of sap conduction. A recent resurgence of interest in this field may lead to a fundamental reassessment of the function of phloem pressure in transport physiology.
PHLOEM PRESSURE AND THE MÜNCH HYPOTHESIS: THEORY
According to Münch (1927) , long-distance transport is driven by osmotically generated hydrostatic pressure (phloem pressure). Photoassimilates and other solutes enter the source end of the sieve tube and attract water by osmosis. The primary sources are mature leaves. Water and solutes exit in sinks, such as roots and meristematic tissues, resulting in bulk flow of solution.
Theoretical treatments of the Münch hypothesis have long suggested that it adequately explains transport over short distances, but not over the distances encountered in tall trees (see Thompson and Holbrook, 2003a ; Jensen et al., 2009b ). Recently, Mullendore et al. (2010) readdressed this issue, calculating the expected pressure drop in several species from detailed data on sieve tube anatomy and axial flow velocity. According to their calculations, based on the model of Thompson and Holbrook (2003a) , values for specific conductivity ( k ) in sieve tubes are limited up to 85% by sieve pores and vary by more than 2 orders of magnitude in different plants. Cucurbits, with their large sieve elements and wide sieve pores, have exceptionally high k values (53 μm 2 ; Mullendore et al., 2010 ), likely as an adaptation to the vine habit. In some species with especially narrow pores, such as Arabidopsis ( Arabidopsis thaliana ), k values are as low as 0.22 μm 2 . The fact that conductance is very high in cucurbits is important in the sense that it sets an attainable upper limit for comparative purposes with other plants.
The expected pressure drop (Δ p ) along the sieve tube equals Uηk −1 ( Mullendore et al., 2010 ) where U is axial flow velocity and η is sap viscosity. The expected Δ p is 0.008 MPa m −1 in Cucurbita maxima and 1.0 MPa m −1 in Arabidopsis. For the purpose of these calculations it is presumed that the sieve pores are open and free of cytoplasm, a reasonable assumption in angiosperms ( Knoblauch and Van Bel, 1998 ), but not in gymnosperms ( Schulz, 1992 ).
With these theoretical calculations in hand, we can now see if there is enough measured pressure in sieve tubes to drive flow. One clear expectation is that, other factors being equal, the sieve tubes of trees should have higher turgor than those of herbaceous plants.
PHLOEM PRESSURE DOES NOT SCALE TO PLANT SIZE
Data do not support the prediction that phloem pressure scales to plant size. Indeed, the opposite appears to be true. It must be kept in mind that these measurements are difficult to make since sieve elements are narrow and secluded in complex tissue. The most reliable method is to take advantage of an aphid’s ability to insert its stylet into a sieve element without disrupting flow. Pressure is measured directly by sealing a micropipette over the severed stylet ( Wright and Fisher, 1980 ). Phloem pressure can also be measured with a probe ( Hammel, 1968 ), or calculated from measurements of osmotic and water potentials (Sovonick-Dunford et al., 1981).
Strikingly, the lowest measured values of phloem pressure (0.6–1.4 MPa) are from trees ( Hammel, 1968 ; Wright and Fisher, 1980 ; Lee, 1981 ; Sovonick-Dunford et al., 1981 ). Not all of this pressure is available to drive flow since pressure in sink sieve elements in many organs is at least equal to that of surrounding cells, approximately 0.7 MPa (e.g. Pritchard, 1996 ). This leaves at most 0.7 MPa to drive transport. Several studies from willow ( Salix spp.) indicate phloem pressure of approximately 0.7 MPa, which does not allow for any gradient. Lee (1981) measured significant differences in turgor at various elevations in ash ( Fraxinus americana ), but in some cases it was actually higher close to the roots, and Zimmermann’s (1957) measurements of sugar gradients in ash can only be reconciled with theory if the pressure in the phloem of the roots is zero.
The issue of phloem pressure in gymnosperms, some of which transport over exceptionally long distances, is especially acute. In Pinus strobus , k = 4.4 μm 2 ( Thompson and Holbrook, 2003b ), which is similar to that of Phaseolus vulgaris , assuming that the pores are open. However, there is no way to reconcile the data indicating plugged sieve pores ( Schulz, 1992 ) with Münch pressure flow.
To deepen the mystery, it appears that many tree species do not take advantage of active phloem loading mechanisms to elevate pressure at the source ends of the sieve tubes ( Reidel et al., 2009 ; Rennie and Turgeon, 2009 ). Two active loading mechanisms have been described: uptake from the apoplast energized by the proton motive force, and polymer trapping ( Slewinski and Braun, 2010 ; Turgeon, 2010 ). However, in some species, almost all of which are trees, loading appears to be passive, with no uphill Suc gradient from mesophyll to phloem ( Reidel et al., 2009 ; Rennie and Turgeon, 2009 ).
It could be argued that turgor measured in stems of trees appears low because it is measured distantly from the leaves in which it is generated. However, osmotic potentials in minor vein sieve elements of poplar ( Populus deltoides ; Russin and Evert, 1985 ) and willow ( Turgeon and Medville, 1998 ) are considerably lower than those of herbaceous plants.
The analysis above leaves the impression that trees live at the extreme edge of transport capability, with phloem transport motivated by a barely sufficient driving force. However, if this were so, sieve tube conductivity should have evolved to a maximum. It has not. The diameters of sieve pores, the sites of most resistance to flow, are similar in the trees that have been examined to those of most herbs, and well below those of cucurbits ( Thompson and Holbrook, 2003b ). Photoassimilate travels down the stems to the roots of angiosperm trees at velocities that rival those of herbaceous plants ( Plain et al., 2009 ).
There are two obvious explanations for the discrepancy between theory and data as they pertain to trees. The first is that the discrepancy is more apparent than real. It is possible that technical limitations have led to an underestimation of phloem pressure and conductivity and an overestimation of sap velocity. It is also possible that advances in the theory of flow through tubes with the dimensions and biological attributes of living phloem may lead to a reconciliation between measurements and calculations. In this regard, techniques in microfluidics may better approximate reality than the more macroscopic approaches used to date ( Jensen et al., 2009a ).
The second possibility is that we are missing something fundamental. For example, it has been suggested that the phloem consists of relays or functional units and that solutes are transported energetically from one unit to the next, providing a boost in pressure at intervals along the transport pathway ( Lang, 1979 ; Aikman, 1980 ).
The difficulty with the relay hypothesis is that phloem sap is rich in organic molecules and ions ( Turgeon and Wolf, 2009 ). Transfer across the plasma membrane would require an elaborate set of transporters unless the composition of the sap changes at each step. The long-distance transport of macromolecules ( Dinant and Lemoine, 2010 ) poses a special problem, if they are indeed transported over the entire dimensions of a tree. This issue might not be as pertinent if the relay operates by electroosmosis. As difficult as the relay hypothesis may be to verify, it deserves further attention.
Data are more hospitable to Münch pressure flow in herbs. Phloem turgor is high, even though transport distances are short. For example, pressure readings from aphid stylets indicate turgor of 2.4 MPa in wheat ( Triticum aestivum ; Fisher and Cash-Clark, 2000 ) and 1.9 MPa in Sonchus oleraceus ( Gould et al., 2004 ). For a Δ p as high as 0.5 MPa m −1 , there is more pressure than necessary to drive translocation. Perhaps the best demonstration of a gradient (0.2 MPa m −1 osmotic potential) comes from Nicotiana glauca ( Hocking, 1980 ). If one assumes a water potential gradient of 0.1 MPa m −1 in the opposite direction, this would indicate a Δ p of 0.1 MPa m −1 in the direction of flow.
Pressure gradients are difficult or impossible to detect in smaller plants ( Fisher and Gifford, 1986 ; Pritchard, 1996 ). Indeed, it is striking that available pressure is so much in excess of any detectable Δ p between source and sink phloem. In sugar beet ( Beta vulgaris ), the osmotic pressure in importing veins of sink leaves (2.27 MPa) is only 10% less than in source leaf veins (2.52 MPa; Fellows and Geiger, 1974 ). The excessive turgor in the phloem of small plants suggests that this pressure has additional functions especially suited to the herbaceous growth habit.
WHY IS THE PHLOEM PRESSURIZED?
An obvious advantage to high phloem pressure is that it defends plants. When a sieve tube is wounded, pressure release causes surging and forces cellular debris into the sieve pores, sealing the phloem. Some insects have adapted and can maintain flow with compounds in their saliva ( Will et al., 2007 ), but the majority are confounded by this blunt but effective strategy.
Phloem pressure is also an important factor in regulation. Fisher (2000) describes the phloem as a high-pressure manifold distribution system from which assimilates are bled out of a low-resistance pathway (the sieve tube) by high-resistance leaks (plasmodesmata). This results in uniformity of unloading potential throughout the plant. The principle is apparent in households: Flow from faucets in different parts of a house is similar when pressure in the pipes is high, but differs annoyingly from one faucet to another if pressure drops.
Thompson and Holbrook (2003b) also argue that integrative control over the whole plant is most effective when osmotic strength in sieve tubes is high and Δ p along the axis is small. Under these conditions, localized unloading in a sink automatically reduces turgor everywhere and provides a signal to load more sugar ( Patrick, 1994 ; Thompson, 2006 ).
Elevated pressure also minimizes the effect of reduced water potential in times of water stress ( Smith and Milburn, 1980 ). The advantages in maintaining an uninterrupted supply of nutrients and phloem water to meristematic zones, and the pressure needed for effective regulation, are obvious.
Another way to view excessively high phloem pressure is that it is a secondary consequence of active loading, not its primary purpose. Active loading increases the concentration of transport solute in the sieve tubes, discouraging phloem feeders by creating osmotic potential high enough to desiccate animal tissues. Aphids have strategies to reduce the osmotic potential of the sap in their gut ( Ashford et al., 2000 ), but not all insects have this capability. Also, by increasing the soluble carbohydrate content in sieve tubes, the amount of carbon and energy delivered per unit volume rises and the amount of excess water that has to be dealt with by sinks, especially dry seeds and fruits, is minimized. The trade-off is that viscosity increases with concentration, reducing flux. Lang (1978) calculated that the optimum Suc concentration is 0.75 m , a level that can only be achieved by active loading.
Another advantage to active phloem loading is that it allows plants to reduce inventory of nonstructural carbohydrates in leaves without compromising export ( Turgeon, 2010 ). This strategy frees up reduced carbon compounds to make new leaves. Decreasing unnecessary inventory increases relative growth rate by accelerating formation of new leaves. It is possible that active loading evolved along with the herbaceous growth habit to reduce foliar inventory, resulting in more rapid growth and, incidentally, the additional benefits described above.
The fact that we are still puzzled by fundamental aspects of phloem transport physiology testifies to the subtleties of the mechanisms involved and the technical difficulties associated with conducting experiments on such a reclusive and unaccommodating tissue. A continuing problem in this field is that only fragmentary information is available for individual species and we are therefore forced into making generalities about an ideal plant when it is clear that each parameter, such as sieve pore width, is part of a species-specific adaptive strategy. More comprehensive studies on the physiology and quantitative anatomy of selected species are called for ( Thompson and Wolniak, 2008 ; Mullendore et al., 2010 ), recognizing that there may be fundamental differences in underlying processes in different plant types, especially trees and herbs. Gymnosperms are particularly puzzling in many respects and deserve more attention. Available data suggest that the amount of phloem pressure, and the anatomical parameters that define conductivity, have been evolutionarily optimized in both woody and herbaceous plants for several purposes, only one of which is to motivate long-distance transport.
Acknowledgments
I thank Michael Knoblauch, John Patrick, Thomas Slewinski, and Michael Thorpe for constructive reviews of the manuscript.
- Aikman D. (1980) Contractile proteins and hypotheses concerning their role in phloem transport . Can J Bot 58 : 826–832 [ Google Scholar ]
- Ashford DA, Smith WA, Douglas AE. (2000) Living on a high sugar diet: the fate of sucrose ingested by a phloem-feeding insect, the pea aphid Acyrthosiphon pisum . J Insect Physiol 46 : 335–341 [ PubMed ] [ Google Scholar ]
- Dinant S, Lemoine R. (2010) The phloem pathway: new issues and old debates . C R Biol 333 : 307–319 [ PubMed ] [ Google Scholar ]
- Fellows RJ, Geiger DR. (1974) Structural and physiological changes in sugar beet leaves during sink to source conversion . Plant Physiol 54 : 877–885 [ PMC free article ] [ PubMed ] [ Google Scholar ]
- Fisher DB. (2000) Long-distance transport . Buchanan BB, Gruissem W, Jones RL, , Biochemistry and Molecular Biology of Plants . American Society of Plant Physiologists, Rockville, MD, pp 730–784 [ Google Scholar ]
- Fisher DB, Cash-Clark CE. (2000) Sieve tube unloading and post-phloem transport of fluorescent tracers and proteins injected into sieve tubes via severed aphid stylets . Plant Physiol 123 : 125–137 [ PMC free article ] [ PubMed ] [ Google Scholar ]
- Fisher DB, Gifford RM. (1986) Accumulation and conversion of sugars by developing wheat grains VI. Gradients along the transport pathway from the peduncle to the endosperm cavity during grain filling . Plant Physiol 82 : 1024–1030 [ PMC free article ] [ PubMed ] [ Google Scholar ]
- Gould N, Minchin PEH, Thorpe MR. (2004) Direct measurements of sieve element hydrostatic pressure reveal strong regulation after pathway blockage . Funct Plant Biol 31 : 987–993 [ PubMed ] [ Google Scholar ]
- Hammel HT. (1968) Measurement of turgor pressure and its gradient in the phloem of oak . Plant Physiol 43 : 1042–1048 [ PMC free article ] [ PubMed ] [ Google Scholar ]
- Hocking PJ. (1980) The composition of phloem exudate and xylem sap from tree tobacco ( Nicotiana glauca Grah.) . Ann Bot (Lond) 45 : 633–643 [ Google Scholar ]
- Jensen KH, Lee J, Bohr T, Bruus H. (2009a) Osmotically driven flows in microchannels separated by a semipermeable membrane . Lab Chip 9 : 2093–2099 [ PubMed ] [ Google Scholar ]
- Jensen KH, Rio E, Hansen R, Clanet C, Bohr T. (2009b) Osmotically driven pipe flows and their relation to sugar transport in trees . J Fluid Mech 636 : 371–396 [ Google Scholar ]
- Knoblauch M, Van Bel AJE. (1998) Sieve tubes in action . Plant Cell 10 : 35–50 [ Google Scholar ]
- Lang A. (1978) A model of mass flow in the phloem . Aust J Plant Physiol 5 : 535–546 [ Google Scholar ]
- Lang A. (1979) A relay mechanism for phloem translocation . Ann Bot (Lond) 44 : 141–145 [ Google Scholar ]
- Lee D. (1981) Synchronous pressure-potential changes in the phloem of Fraxinus americana L . Planta 151 : 304–308 [ PubMed ] [ Google Scholar ]
- Mullendore D, Windt C, Van As H, Knoblauch M. (2010) Sieve tube geometry in relation to phloem flow . Plant Cell 22 : 579–593 [ PMC free article ] [ PubMed ] [ Google Scholar ]
- Münch E. (1927) Versuche über den Saftkreislauf . Ber Dtsch Bot Ges 45 : 340–356 [ Google Scholar ]
- Patrick J. (1994) Turgor-dependent unloading of assimilates from coats of developing legume seed: assessment of the significance of the phenomenon in the whole plant . Physiol Plant 90 : 645–654 [ Google Scholar ]
- Plain C, Gerant D, Maillard P, Dannoura M, Dong Y, Zeller B, Priault P, Parent F, Epron D. (2009) Tracing of recently assimilated carbon in respiration at high temporal resolution in the field with a tuneable diode laser absorption spectrometer after in situ 13 CO 2 pulse labelling of 20-year-old beech trees . Tree Physiol 29 : 1433–1445 [ PubMed ] [ Google Scholar ]
- Pritchard J. (1996) Aphid stylectomy reveals an osmotic step between sieve tube and cortical cells in barley roots . J Exp Bot 47 : 1519–1524 [ Google Scholar ]
- Reidel EJ, Rennie EA, Amiard V, Cheng L, Turgeon R. (2009) Phloem loading strategies in three plant species that transport sugar alcohols . Plant Physiol 149 : 1601–1608 [ PMC free article ] [ PubMed ] [ Google Scholar ]
- Rennie EA, Turgeon R. (2009) A comprehensive picture of phloem loading strategies . Proc Natl Acad Sci USA 106 : 14162–14167 [ PMC free article ] [ PubMed ] [ Google Scholar ]
- Russin WA, Evert RF. (1985) Studies on the leaf of Populus deltoides (Salicaceae): ultrastructure, plasmodesmatal frequency, and solute concentrations . Am J Bot 72 : 1232–1247 [ Google Scholar ]
- Schulz A. (1992) Living sieve cells of conifers as visualized by confocal, laser-scanning fluorescence microscopy . Protoplasma 166 : 153–164 [ Google Scholar ]
- Slewinski TL, Braun DM. (2010) Current perspectives on the regulation of whole-plant carbohydrate partitioning . Plant Sci 178 : 341–349 [ Google Scholar ]
- Smith JAC, Milburn JA. (1980) Phloem turgor and the regulation of sucrose loading in Ricinus communis L . Planta 148 : 42–48 [ PubMed ] [ Google Scholar ]
- Sovonick-Dunford S, Lee D, Zimmermann M. (1981) Direct and indirect measurements of phloem turgor pressure in white ash . Plant Physiol 68 : 121–126 [ PMC free article ] [ PubMed ] [ Google Scholar ]
- Thompson M, Holbrook N. (2003a) Application of a single-solute non-steady-state phloem model to the study of long-distance assimilate transport . J Theor Biol 220 : 419–455 [ PubMed ] [ Google Scholar ]
- Thompson M, Holbrook N. (2003b) Scaling phloem transport: water potential equilibrium and osmoregulatory flow . Plant Cell Environ 26 : 1561–1577 [ Google Scholar ]
- Thompson M, Wolniak S. (2008) A plasma membrane-anchored fluorescent protein fusion illuminates sieve element plasma membranes in Arabidopsis and tobacco . Plant Physiol 146 : 1599–1610 [ PMC free article ] [ PubMed ] [ Google Scholar ]
- Thompson MV. (2006) Phloem: the long and the short of it . Trends Plant Sci 11 : 26–32 [ PubMed ] [ Google Scholar ]
- Turgeon R. (2010) The role of phloem loading reconsidered . Plant Physiol 152 : 1817–1823 [ PMC free article ] [ PubMed ] [ Google Scholar ]
- Turgeon R, Medville R. (1998) The absence of phloem loading in willow leaves . Proc Natl Acad Sci USA 95 : 12055–12060 [ PMC free article ] [ PubMed ] [ Google Scholar ]
- Turgeon R, Wolf S. (2009) Phloem transport: cellular pathways and molecular trafficking . Annu Rev Plant Biol 60 : 207–221 [ PubMed ] [ Google Scholar ]
- Will T, Tjallingii WF, Thonnessen A, van Bel AJE. (2007) Molecular sabotage of plant defense by aphid saliva . Proc Natl Acad Sci USA 104 : 10536–10541 [ PMC free article ] [ PubMed ] [ Google Scholar ]
- Wright JP, Fisher DB. (1980) Direct measurement of sieve tube turgor pressure using severed aphid stylets . Plant Physiol 65 : 1133–1135 [ PMC free article ] [ PubMed ] [ Google Scholar ]
- Zimmermann M. (1957) Translocation of organic substances in trees. II. On the translocation mechanism in the phloem of white ash ( Fraxinus americana L.) . Plant Physiol 32 : 399–404 [ PMC free article ] [ PubMed ] [ Google Scholar ]
Thank you for visiting nature.com. You are using a browser version with limited support for CSS. To obtain the best experience, we recommend you use a more up to date browser (or turn off compatibility mode in Internet Explorer). In the meantime, to ensure continued support, we are displaying the site without styles and JavaScript.
- View all journals
- Explore content
- About the journal
- Publish with us
- Sign up for alerts
- News & Views
- Published: 21 February 2022
PHLOEM LOADING
Low sugar, under pressure?
- Li-Qing Chen ORCID: orcid.org/0000-0002-0964-5388 1
Nature Plants volume 8 , pages 102–103 ( 2022 ) Cite this article
1075 Accesses
12 Altmetric
Metrics details
- Plant physiology
- Plant transporters
The difference in phloem pressure is the driving force that moves photosynthetic products and other solutes from the source to the sink. Measurements with emerging technologies reveal that sugar loading is not essential for maintaining phloem pressure and phloem bulk flow in the maize sugar-loading-defective mutant sut1 .
This is a preview of subscription content, access via your institution
Access options
Access Nature and 54 other Nature Portfolio journals
Get Nature+, our best-value online-access subscription
24,99 € / 30 days
cancel any time
Subscribe to this journal
Receive 12 digital issues and online access to articles
111,21 € per year
only 9,27 € per issue
Buy this article
- Purchase on Springer Link
- Instant access to full article PDF
Prices may be subject to local taxes which are calculated during checkout
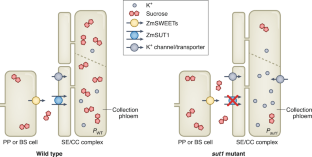
Münch, E. Die Stoffbewegungen in der Pflanze (G. Fischer, 1930).
Knoblauch, M. et al. eLife 5 , e15341 (2016).
Article Google Scholar
Patrick, J. W. & Tyerman, S. D. in Biochemistry and Molecular Biology of Plants (eds. Buchanan, B. et al.) 658–710 (Wiley, 2015).
Babst, B. A. et al. Nat. Plants https://doi.org/10.1038/s41477-022-01098-x (2021).
Chen, L. Q. New Phytol. 201 , 1150–1155 (2014).
Article CAS Google Scholar
Baker, R. F. et al. Plant Physiol. 172 , 1876–1898 (2016).
Bezrutczyk, M. et al. New Phytol. 218 , 594–603 (2018).
Knoblauch, J., Mullendore, D. L., Jensen, K. H. & Knoblauch, M. Plant Physiol. 166 , 1271–1279 (2014).
Kopittke, P. M. et al. Plant Physiol. 178 , 507–523 (2018).
Edelman, A., Chanson, M. & Hug, M. J. J. Cyst. Fibros. 3 (Suppl. 2), 113–117 (2004).
Dreyer, I., Gomez-Porras, J. L. & Riedelsberger, J. New Phytol. 216 , 1049–1053 (2017).
Download references
Author information
Authors and affiliations.
Department of Plant Biology, University of Illinois at Urbana-Champaign, Urbana, IL, USA
Li-Qing Chen
You can also search for this author in PubMed Google Scholar
Corresponding author
Correspondence to Li-Qing Chen .
Ethics declarations
Competing interests.
The authors declare no competing interests.
Rights and permissions
Reprints and permissions
About this article
Cite this article.
Chen, LQ. Low sugar, under pressure?. Nat. Plants 8 , 102–103 (2022). https://doi.org/10.1038/s41477-021-01034-5
Download citation
Published : 21 February 2022
Issue Date : February 2022
DOI : https://doi.org/10.1038/s41477-021-01034-5
Share this article
Anyone you share the following link with will be able to read this content:
Sorry, a shareable link is not currently available for this article.
Provided by the Springer Nature SharedIt content-sharing initiative
Quick links
- Explore articles by subject
- Guide to authors
- Editorial policies
Sign up for the Nature Briefing newsletter — what matters in science, free to your inbox daily.

- Share full article
Advertisement
Supported by
What Happens if a Powerful Surveillance Law Expires This Week?
Senators are under pressure to reject amendments to a House-passed bill so it can become law before a statute expires Friday night. But the program would continue after any such lapse — with some caveats.

By Charlie Savage
Charlie Savage has been writing about national security legal policy, including surveillance, for more than two decades. He reported from Washington.
Senate leaders of both parties are urging their colleagues to renew an expiring warrantless surveillance law before it lapses at midnight on Friday, as advocates of the law have argued that any expiration would mean going blind on a key source of counterterrorism information and other foreign intelligence.
That deadline adds pressure to senators not to vote for any amendments to the version of the bill that the House passed last week , since any changes would force the legislation to go back to the House rather than swiftly arriving on President Biden’s desk.
But the suggestion that the tool itself would simply lapse on April 19 is significantly misleading. A national security court this month granted a request from the government that allows the program to operate for another year, even if the law, known as Section 702, expires. Still, it is true that such an expiration could lead to smaller gaps in collecting some messages.
Here is a closer look.
What is Section 702?
It is a law that authorizes the government to collect, without a warrant and from U.S. companies like AT&T and Google, messages of foreigners abroad who are targeted for intelligence or counterterrorism purposes.
The idea is that in the internet era, foreigners’ communications are often handled by domestic companies. But it is controversial because the government also sweeps up messages of Americans to and from those foreign targets.
The law traces back to a warrantless wiretapping program that President George W. Bush secretly created after the terrorist attacks of Sept. 11, 2001. It violated the Foreign Intelligence Surveillance Act, or FISA, which requires warrants for national security wiretapping on domestic soil.
After the program came to light, Congress in 2007 legalized a form of it in a short-lived law called the Protect America Act. Lawmakers enacted Section 702 the next year, carving out a more enduring exception to FISA. Congress extended Section 702 in 2012 and 2018. It is now set to expire again.
What are the proposals before the Senate?
The House bill would tighten some controls on Section 702, while extending it for another two years. The bill would also expand the program in several ways, including by allowing it to be used to scrutinize foreign drug cartels.
While Senator Chuck Schumer, Democrat of New York and the majority leader, has not yet announced the details, it appears likely that before voting on that bill, the Senate will consider several proposed amendments sought by surveillance skeptics and reform-minded lawmakers.
Among them will probably be a proposal to bar officials from searching the repository of messages collected under Section 702 for the content of communications by Americans unless the government first obtains a warrant. Privacy advocates have long sought such a change, while national security officials strongly oppose it, saying it would cripple the program. A similar amendment in the House failed in a 212-to-212 tie vote .
Another possible amendment would remove an enigmatically worded provision the House added to the bill that expands the type of service providers that can be compelled to participate in the program. The provision is aimed at certain data centers for cloud computing that the FISA court ruled in 2022 fell outside the current definition of what services the statute covers, according to people familiar with the matter.
Privacy advocates have warned that it is too broadly worded, leaving open the potential for abuses. On Thursday, the Justice Department sent a letter to Congress committing to using the expanded definition “exclusively to cover the type of service provider at issue” in the 2022 litigation and pledging to report to Congress every six months about its use. The letter also said “the number of technology companies providing this service is extremely small.”
The Senate could also vote on a proposal to bar the government from purchasing personal information about Americans from third-party data brokers that it would need a warrant to obtain directly from a company. The House on Wednesday voted to approve a stand-alone bill containing that measure, called the Fourth Amendment is Not For Sale Act .
Why would the program continue if its law has expired?
Congress wrote into the 2008 law a provision ensuring that the government would not be abruptly cut off from using the Section 702 program.
The program operates under certifications issued each year by the Foreign Intelligence Surveillance Court, and the government directs communications companies to participate in it. Crucially, the provision, Section 404(b), says that despite anything else in the statute, these orders or directives “shall continue in effect” until their expiration dates.
Because the FISA court issued a round of certifications this month that expire next April 4, this provision appears to mean that the Section 702 program can lawfully keep operating until then, even if Congress allows the underlying statute to lapse in the meantime.
Has this theory been legally tested?
In April 2008, the presiding judge of the FISA court at the time, Reggie B. Walton, ruled that a similar provision in Section 702’s precursor law, the Protect America Act, meant that a directive to Yahoo still had legal force after the act itself had expired — and that the court could still compel Yahoo to comply with it.
It is clear, Judge Walton wrote , “that, even after that expiration date, the challenged directives ‘remain in effect until their expiration.’”
That August, a panel of three federal appeals court judges upheld Judge Walton’s ruling . Those precedents suggest the FISA court would rule the same way about Section 702.
Does that mean there is no risk of a gap in collecting communications?
While the overall program would continue, if the statute’s lapse prompts a particular provider to balk at cooperating, there could be at least a temporary pause in collection from that entity, according to a senior Justice Department official.
The matter would end up in court, as happened with Yahoo in 2008. Even if the government ultimately prevailed, there could be a gap in collection of communications from that company. It is not clear how swiftly the court would resolve such a case.
What kind of dispute does the Justice Department think it could win?
The department believes it could win a dispute in which a program participant balks at continuing to fully cooperate after the statute lapses.
Under some iterations of this scenario, a company might stop turning over all communications of targeted foreign users. Or it might keep turning over those the government had ordered it to target before the lapse but balk at adding any new ones.
The senior Justice Department official said the agency was confident that the government would prevail before the FISA court in such a fight, citing the Yahoo precedent. The official, who spoke on the condition of anonymity to discuss a legally sensitive topic, also noted that the directives are explicitly written to anticipate that the government would provide new targets over time.
What might the government not be allowed to do?
The government may be prohibited from forcing a new service to start participating in the program.
While major communications companies already participate, new internet-based communication services regularly emerge. When agencies learn that a suspected adversary is using a service that is not part of the program, the government directs it to join the program. According to the Justice Department official, this happens multiple times a year.
If the provider balks, the matter goes to the FISA court. But since the provision centers on allowing orders that were already in effect to continue until they themselves expire, it is not clear that the executive branch or the court would have the power to issue new orders to a new service.
Charlie Savage writes about national security and legal policy. More about Charlie Savage
A Divided Congress: Latest News and Analysis
House Approves $95 Billion Aid: The House voted resoundingly to approve $95 billion in foreign aid for Ukraine, Israel and Taiwan, as Speaker Mike Johnson put his job on the line to advance the long-stalled aid package by marshaling bipartisan support. Here’s the breakdown and how the House voted .
Extension of Surveillance Law: The Senate approved an extension of a warrantless surveillance law, sending President Biden legislation that national security officials say is crucial to fighting terrorism but that privacy advocates decry as a threat to Americans’ rights.
A Divided G.O.P.: The House vote on Ukraine aid was the clearest sign yet that at least on foreign policy, the Republican Party is not fully aligned with former President Donald J. Trump and his “America First” movement .
TikTok Bill: The House made another push to force through legislation that would require the sale of TikTok by its Chinese owner or ban the app in the United States by packaging the measure with aid to Ukraine and Israel .
Mayorkas Impeachment: Republicans say the Senate’s quick dismissa l of charges against Alejandro Mayorkas, the homeland security secretary, sets a dangerous precedent. Democrats say the mistake would have been to treat the case seriously .
Campus Antisemitism Hearing: Columbia’s president, Nemat Shafik, agreed that the university needed to take a tougher stance on antisemitism, in response to harsh questioning from a Republican-led House committee .

IMAGES
VIDEO
COMMENTS
The pressure flow hypothesis, also known as the mass flow hypothesis, is the best-supported theory to explain the movement of sap through the phloem. [1] [2] It was proposed by Ernst Münch, a German plant physiologist in 1930. [3] A high concentration of organic substances, particularly sugar, inside cells of the phloem at a source, such as a ...
The Pressure-Flow Hypothesis Figure 16.2.2.3 Pressure flow. The best-supported theory to explain the movement of food through the phloem is called the pressure-flow hypothesis.. It proposes that water containing food molecules flows under pressure through the phloem.
In brief, according to the Münch pressure flow hypothesis (Knoblauch and Peters, 2013) solutes are loaded into the sieve tubes of leaves, and water is moved osmotically into the sieve tubes, creating a positive internal pressure. As sucrose and other sugars, together with amino acids and ureides in some species, are the dominant osmotically ...
Figure 16.2.2.3 Pressure flow. The best-supported theory to explain the movement of food through the phloem is called the pressure-flow hypothesis. It proposes that water containing food molecules flows under pressure through the phloem. The pressure is created by the difference in water concentration of the solution in the phloem and the ...
As a result, water moves from the phloem by osmosis and is then transpired or recycled via the xylem back into the phloem sap. Figure 4.5.2.4 4.5.2. 4: The pressure-flow hypothesis of assimilate transport. The assimilate, which is rich in sucrose, is actively transported from source cells into companion cells and then into the sieve-tube ...
The Pressure Flow Model for Sugar Translocation Requires the Movement of Water into and out of the Phloem. The information below was adapted from OpenStax Biology 30.5. The best-supported hypothesis to explain the movement of sugars in phloem is the pressure flow model for phloem transport. This hypothesis accounts for several observations:
#biologyanimation The pressure-flow hypothesis explains why phloem sapflows from source to sink, and experiments build a strongcase for pressure flow as the ...
The pressure-flow hypothesis is the most well-supported theory explaining how nutrients in sap are transported throughout the phloem of the plant, i.e., from photosynthetic sources to sinks. This ...
One possibility is the 'pressure flow hypothesis' proposed by the German plant physiologist Ernst Münch in 1930. By making careful measurements of the physical properties of morning glory ...
The two most discussed potential modes of translocation were the electro-osmotic theory (Fensom, 1957; Spanner, 1958, 1970) and the pressure-flow hypothesis (Münch, 1927, 1930). The electro-osmotic theory required sieve plate pores to be filled with material. Potassium pumps were suggested to generate a circulation of ions through the plate.
The pressure flow hypothesis introduced by Ernst Mu¨nch in 1930 describes a mechanism of osmotically generated pressure differentials that are supposed to drive the movement of sugars and other solutes in the phloem, but this hypothesis has long faced major challenges. The key issue is
Now, how this flow, um, sap is going to move through the flow. Um, is explained by the pressure flow hypothesis. This is the most commonly agreed upon theory for the movement of sap through flow. Um, essentially, the idea is this sugar is going to be more concentrated at the source, right.
According to his theory, the mass flow in the phloem is driven by an osmotically generated pressure gradient. As the sieve pores interconnect the protoplasts of the sieve tubes, the transport in the sieve tube itself is a mass flow driven by a pressure (or turgor) gradient. ... As such, the Münch pressure flow seems more suitable for herbs ...
The pressure flow hypothesis introduced by Ernst Münch in 1930 describes a mechanism of osmotically generated pressure differentials that are supposed to drive the movement of sugars and other solutes in the phloem, but this hypothesis has long faced major challenges. The key issue is whether the conductance of sieve tubes, including sieve ...
The Mass Flow Hypothesis was first proposed by German plant physiologist Ernst Munch in the year 1930. He theorised the movement of sap through the phloem tissue in plants. This theory is also known as the Pressure Flow Hypothesis. A highly concentrated organic sugar especially sugar in the cells of the phloem from a source like a leaf forms a ...
The pressure-flow hypothesis predicts pressure and sap flow velocity in sut1 mutants when both sucrose and K + are included as osmolytes, but not sucrose alone.
The Pressure Flow Hypothesis, also known as the Mass Flow Hypothesis, is the best-supported theory to explain the movement of sap through the phloem. It was proposed by Ernst Munch, a Germany plant physiologist in 1930. A high concentration of organic substances, particularly sugar, inside cells of the phloem at a source, such as a leaf ...
A major problem is that it is difficult to reconcile the theory of pressure flow, proposed over 80 years ago by Ernst Münch (1927), with transport in large trees. Significantly, phloem pressure does not scale to plant size. Also, the very high turgor in the phloem of herbs suggests that pressure has functions beyond that of sap conduction.
The pressure-flow hypothesis often referred to as the mass flow hypothesis, is the best-supported explanation for sap transportation through the phloem. It was proposed by Ernst Münch, a German plant physiologist, in 1930. A diffusion gradient (osmotic gradient) is created when a high concentration of organic compounds, particularly sugar ...
Long-distance phloem transport in plants is proposed to be driven by osmotically generated hydrostatic pressure. This widely accepted pressure-flow model was introduced by Ernst Münch 1 in 1930 ...
We suggest that had this not been so, an earlier version of the pressure-flow hypothesis of photo-assimilate transport probably would have gained traction many decades before Münch's version did. CIRCULATING JUICES. The cambium is the meristematic tissue located between xylem and phloem that is responsible for secondary thickening in woody plants.
Other articles where pressure-flow hypothesis is discussed: angiosperm: Process of phloem transport: Mass-flow hypotheses include the pressure-flow hypothesis, which states that flow into sieve tubes at source regions (places of photosynthesis or mobilization and exportation of storage products) raises the osmotic pressure in the sieve tube; removal of sugars from sieve tubes in sink regions ...
The pressure that Bernoulli's principle is referring to is the internal fluid pressure that would be exerted in all directions during the flow, including on the sides of the pipe. This is different from the pressure a fluid will exert on you if you get in the way of it and stop its motion.
Senators are under pressure to reject amendments to a House-passed bill so it can become law before a statute expires Friday night. But the program would continue after any such lapse — with ...