Research Areas
Research activity in the Biology Department spans the full range of biological organization, from molecules to ecosystems. Main research fields are indicated here, as links to groups of faculty doing research in those areas.


Biochemistry

Cell Biology

Computational Biology

Conservation Biology

Developmental Biology

Marine Biology

Microbiology

Molecular Biology

Neurobiology

Plant Biology

Population Biology
Support Biology
Dei council and dei faculty committee, biology diversity community, mit biology catalyst symposium, honors and awards, employment opportunities, faculty and research, current faculty, in memoriam, areas of research, biochemistry, biophysics, and structural biology, cancer biology, cell biology, computational biology, human disease, microbiology, neurobiology, stem cell and developmental biology, core facilities, video gallery, faculty resources, undergraduate, why biology, undergraduate testimonials, major/minor requirements, general institute requirement, advanced standing exam, transfer credit, current students, subject offerings, research opportunities, biology undergraduate student association, career development, why mit biology, diversity in the graduate program, nih training grant, career outcomes, graduate testimonials, prospective students, application process, interdisciplinary and joint degree programs, living in cambridge, graduate manual: key program info, graduate teaching, career development resources, biology graduate student council, biopals program, postdoctoral, life as a postdoc, postdoc associations, postdoc testimonials, workshops for mit biology postdocs entering the academic job market, responsible conduct of research, postdoc resources, non-mit undergraduates, bernard s. and sophie g. gould mit summer research program in biology (bsg-msrp-bio), bsg-msrp-bio gould fellows, quantitative methods workshop, high school students and teachers, summer workshop for teachers, mit field trips, leah knox scholars program, additional resources, mitx biology, biogenesis podcast, biology newsletter, department calendar, ehs and facilities, graduate manual, resources for md/phd students, preliminary exam guidelines, thesis committee meetings, guidelines for graduating, mentoring students and early-career scientists, remembering stephen goldman (1962 – 2022).
For over 50 years, we have played a central role in the growth of molecular life sciences and the revolution in molecular and cellular biology, genetics, genomics, and computational biology.

An official website of the United States government
The .gov means it's official. Federal government websites often end in .gov or .mil. Before sharing sensitive information, make sure you're on a federal government site.
The site is secure. The https:// ensures that you are connecting to the official website and that any information you provide is encrypted and transmitted securely.
- Publications
- Account settings
- Browse Titles
NCBI Bookshelf. A service of the National Library of Medicine, National Institutes of Health.
National Academy of Sciences (US) Committee on Research in the Life Sciences. The Life Sciences: Recent Progress and Application to Human Affairs: The World of Biological Research Requirements for the Future. Washington (DC): National Academies Press (US); 1970.
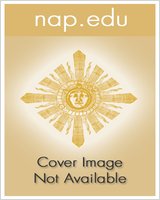
The Life Sciences: Recent Progress and Application to Human Affairs: The World of Biological Research Requirements for the Future.
- Hardcopy Version at National Academies Press
CHAPTER THREE THE WORLD OF BIOLOGICAL RESEARCH
The life sciences embrace a great array of intellectual activity, a continuum extending from the search for the origin of life and the detailed structure of the macromolecules that make life possible to understanding of the total ecology of planet Earth. The millions of micro-organisms and plant and animal species interacting in the air, the soil, freshwater ponds and streams, and the oceans afford a never-ending variety of objects of fascinating inquiry. This endeavor has enhanced man's capacity to manage and protect his environment, to feed and clothe himself, and to prolong his comfortable and fruitful years. The inquiry itself is conducted in the laboratory, in research institutes and hospitals, in experimental tracts and ponds, by walks in the woods, by surveillance from the skies, from ships at sea, and on treks through the jungle, observing both undisturbed and managed nature. Those so engaged range from amateur nature lovers to directors of large institutes. They work in and out of institutions large and small; they work with private, state, and federal resources in institutions of higher learning, nonprofit research institutes, research hospitals, federal, state, and local laboratories, and in the organized multidisciplinary teams of industry.
In 1966 the National Register of Scientific Personnel identified approximately 84,000 individuals with diverse levels of training and educational backgrounds who classified themselves as working life scientists. The identification of these people was possible through the cooperation of the two major biological research societies, the Federation of American Societies for Experimental Biology and the American Institute of Biological Sciences.
The Federation issued questionnaires to approximately 24,300 people, the great majority of whom had earned doctoral degrees. Of these, some 20,100, or 83 percent, responded to the Register questionnaires. The American Institute of Biological Sciences contributed approximately 59,800 names, but the proportion of doctorate holders among this group is lower, and hence fewer of them meet the conditions for inclusion in our survey as individual life scientists. Approximately 40,000 people, or 67 percent, responded to the Institute's questionnaire and the proportion of doctorate holders represented by those respondents is higher than that of the original 59,800 individuals surveyed by that society. The overall response to the Register from the two societies was approximately 65 percent and should comprise most working biologists. From these numbers it can be estimated that 70 to 80 percent of doctoral-degree holders responded to the National Register in 1966. However, one can only guess what fraction of American biologists, with or without doctoral degrees, this represents.
It is estimated * that, in the aggregate, $2,264 million was invested in research in the life sciences in fiscal year 1967, of which 60.3 percent came from the federal government, 7.3 percent from the resources of nonprofit institutions, and 30.0 percent from industry. In its entirety, therefore, research in the life sciences has become one of the major pursuits of American society. This chapter is devoted to a description of some of the components of the life sciences research system, based largely on information gathered from responses to our two questionnaires (Appendixes A and B).
Detailed information on the gross parameters of the total system was revealed by the first of our two questionnaires: It contains 14,362 scientists, of whom 12,383 were investigators as here defined, viz., they devoted more than 20 percent of their time to research. In 1966 they published more than 24,000 original articles, 489 books, 1,100 reviews, and 7,500 in-house reports and other contributions. The universe revealed by the second questionnaire contains 1,256 academic departments with an aggregate continuing staff of 18,608 scientists, with available research funds (direct costs only) totaling $304 million, operating in 325 acres of laboratory space in which they directed the research and training of 23,287 graduate students and 4,695 postdoctoral fellows and were assisted by 24,481 technicians, secretaries, and other personnel.
Of the 14,362 individuals who replied to the individual questionnaire, 3.4 percent were less than 30 years old and 6.3 percent were at least 60 years of age; 36.2 percent ranged from 30 to 39 years; 36.3 percent ranged from 40 to 49 years; and 17.8 percent were in the range 50 to 59 years. This distribution is fairly close to that of the scientific population at large. The average age of the group was 43.2 years, the median 41 to 42 years. Only 5.1 percent of the total population was female.
Every state of the Union was represented in the reporting of birthplaces. New York was represented by the largest number of scientists (1,989); Pennsylvania and Illinois followed with 880 and 855, respectively; and 631 were born in California; in all, 12,439 had been born in the United States, and 1,866 were foreign-born. All but 41 of the foreign-born regarded themselves as permanent residents of the United States at the time of the questionnaire. The foreign-born life scientists had come to our shores from 81 different nations. The major sources were Canada (292), Germany (236), England (162), Taiwan (142), India (97), Austria (89), Hungary (68), Poland (55), and Japan (50).
- WHERE LIFE SCIENTISTS WORK
Two thirds of the 12,383 investigators were employed by institutions of higher learning; as shown in Table 7 , 14 percent were employed by the federal government, 10 percent by industry, and the remaining 10 percent by a variety of nonprofit organizations—e.g., hospitals, clinics, museums, state and local governments—and a few are self-employed. In a general way, this pattern is relatively independent of the field in which these life scientists were trained ( Figure 33 ). With the exception of horticulturists, those trained in the agricultural sciences are more likely to work for the federal government than those trained in any other scientific area. Of the 68 percent who were trained in the basic biological sciences, biochemists are by far the largest single group, constituting 15 percent of the total population of this study, with microbiologists and physiologists 8 percent and 7 percent of the total, respectively. Although, because of their numbers, these groups are predominant on the faculties of institutions of higher education, biochemists, microbiologists, and pharmacologists are also in great demand outside these institutions. Over 40 percent of those trained in these three disciplines operate in nonacademic environments, with all three unusually well represented in the laboratories of industry.
Principal Employment of Life Scientists.
Type of employment of life scientists, by field of doctoral training. (Source: Survey of Individual Life Scientists, National Academy of Sciences Committee on Research in the Life Sciences.)
Of the 17 percent of our population who were originally trained as physicians, one third also obtained Ph.D. degrees. Seventy percent of the M.D.'s are on the faculties of universities, including virtually all the M.D.-Ph.D.'s; rather few research-performing M.D.'s are in industry, but there is unusually high representation in nonprofit institutions, particularly independent hospitals and clinics and public-health organizations. Those trained as physicians constituted 44 percent of the 3,170 reporting members of faculties of medical schools (and these schools corresponded to 39 percent of the total academic population); these were 87 percent of all reporting physicians. The remainder of the medical faculty was drawn largely from among those originally trained in the basic medical sciences; biochemists predominated in this last group (15 percent of the gross total), with major representation also from physiology, microbiology, and pharmacology.
Because of their relatively large total number, those trained in biochemistry are found throughout the system in substantial numbers. Of 1,834 trained biochemists reporting, 59 percent (1,069) were in institutions of higher learning, including 491 in medical schools, 225 on arts and sciences faculties, 126 in agricultural schools, and 37 in liberal arts colleges. Substantial numbers were also found elsewhere: 247 in the federal government, 275 in industry, and 231 in other nonacademic, nonprofit organizations. (The disciplinary designation, “biochemist,” relates only to the field of original doctoral-level training, and not to the area of science in which the scientist is currently working.)
Of the life scientists in our sample employed by institutions of higher learning, slightly less than 5 percent were at liberal arts colleges. Undoubtedly, a much larger fraction of life scientists, particularly botanists and zoologists, are on the faculties of such institutions, but relatively few engage in research on a scale sufficient to have put them within the scope of this study.
The questionnaire addressed to department chairmen yielded an aggregate faculty for all responding departments of 17,172, of whom 3,852 were on faculties of arts and sciences, 3,907 on the faculties of agricultural schools, and 8,915 on the faculties of medical schools. Although the general employment patterns in the two questionnaire files are similar, the discrepancies are of some interest. Whereas 39 percent of all individual respondents were on the faculties of medical schools, 52 percent of the total departmental faculties reported were so employed. To place this in perspective, it should be noted that, of the 1,256 departments represented in the study, 267 are in agricultural schools, 246 in faculties of arts and sciences, and 694 in medical schools. Of the medical departments, 361 were departments of the preclinical and 333 of the clinical segments of medical schools. Undoubtedly, the returns from the chairmen's questionnaire should be taken as a more valid description of the distribution of the faculties of life scientists than that provided by the individual returns.
The 1,689 individual scientists who indicated that they are employed by the federal government appear to represent a large fraction of the senior life scientists in the federal establishment. The major employers of the 1,689 reporting life scientists within the federal establishment are the Departments of Agriculture (36 percent), Health, Education, and Welfare (27 percent), Defense (15 percent), and the Veterans Administration (11 percent). The patterns of employment of scientists in the various biological disciplines reflect the character of the agency missions rather closely. Thus, 84 percent of all those trained in agricultural sciences now in the federal establishment are employed by the Department of Agriculture; 53 percent of all federally employed M.D.'s actively engaged in research work for the Department of Health, Education, and Welfare; 29 percent of the M.D.'s work in the Veterans Administration; and 18 percent of the M.D.'s work in the Department of Defense.
The disciplinary employment patterns in other areas are repeated in the federal establishment: 32 percent of all federal life scientists were trained in the basic medical sciences, varying from 12 percent in the Department of Agriculture to 55 percent in the Department of Defense. Except for the physicians employed by the Department of Health, Education, and Welfare and the Veterans Administration and the agronomists employed by the Department of Agriculture, biochemists again constitute the largest single group of scientists in all federal agencies, ranging from 7 percent in the Department of Agriculture to 16 percent in the Department of Defense, 21 percent in the Department of Health, Education, and Welfare, and 26 percent in the Veterans Administration.
An additional 135 scientists were employed in federal contract research centers, which are managed by educational or other nonprofit organizations. State governments employed 229 life scientists (1.8 percent of the grand total), largely in hospitals or state health departments and their laboratories, and approximately half as many life scientists were found in municipally controlled institutions of the same character. A significant number, 462 scientists (3.7 percent of the total), were employed by nonprofit institutes, foundations, and privately controlled museums.
There are no reliable indicators to determine whether the 1,155 individual respondents who indicated that they are employed in industry constitute either a large or a true sample of the total number of senior life scientists employed in that sector of the economy. Seventy-six percent were employed by manufacturing industries; two thirds of these were in the pharmaceutical industry. Again, those trained in the basic medical sciences predominate: 262 biochemists were the largest group, followed by 178 microbiologists and 107 pharmacologists. The low representation of other disciplines among investigators in industry is somewhat disconcerting. For example, only two embryologists, three anatomists, four cell biologists, four ecologists, eight animal pathologists, 10 biophysicists, 13 botanists, and 25 zoologists reported that they were in the employ of some industrial establishment.
Finally, in this regard, it should be remarked that of the 12,151 life scientists responding, 442 had obtained Ph.D.'s in chemistry and 114 in other fields of the physical sciences (about one half in physics), while 105 individuals were originally educated as psychologists. (No questionnaires were sent to individual practicing research psychologists or to the chairmen of either psychiatry or psychology departments.) The employment distribution of these 662 converts to the life sciences among institutions of higher learning, the federal government, industry, and other organizations was much like that of the groups described earlier.
- MOBILITY OF LIFE SCIENTISTS
Geographic mobility, so prominently a characteristic of American society, is nowhere more evident than in the scientific community. As shown in Table 8 , scientists born in each of the standard census regions can currently be found in each of the other census regions. Presumably, the direction of these migrations is dictated largely by increasing employment opportunities. This is particularly evident in the considerable migration from all other census regions to the Pacific Coast region and the South Atlantic region. Of at least equal interest, however, is the even greater tendency for relocation to regions likely to produce the least “cultural shock.” Not only is there the expected tendency of a substantial fraction of all scientists in all census regions to remain within the states or census regions within which they were born, but the most frequent move from one region to another has been to an adjoining area where life patterns are similar—e.g., from the lower South to the upper South, or within the Midwest.
Migration Patterns of Life Scientists.
For the entire population of life scientists, the average length of employment in the current position was 9.6 years, with the median 6 to 7 years. Fifty-five percent of all respondents had held at least one previous position with a different employer, quite apart from any number of postdoctoral appointments. The average length of employment in that previous position was 4.7 years, and the median was 3 to 4 years. Although 90.5 percent of all such moves had been made after less than 10 years with the previous employer, employment translocation was reported by some scientists even after as long as 40 years with the initial employer.
The pattern of these moves is of interest in itself. Although institutions of higher learning were the principal source of those who entered the employ of the federal government, private industry, and other organizations, in a general way each employing entity in the system also tended to recruit from other institutions in the same category. For example, 36 percent of all those in private industry had been employed by a different corporation, and 19 percent of those now working for an independent hospital or clinic had previously worked for some other independent hospital or clinic.
Two thirds of those who had moved to an institution of higher learning had come from another such institution. Of the remainder, 13 percent had left the federal government, 5 percent private industry, 5 percent other nonprofit organizations, and 8 percent various other state and community institutions. Perhaps the major surprise in these data is the fact that, ignoring graduate and postdoctorate education, institutions of higher learning appeared to be a net importer of scientific employees. Whereas 1,750 individuals whose previous employers had been nonacademic institutions currently were employed by the universities, only 1,260 individuals currently employed by nonacademic institutions had previously been employed by universities or colleges.
Respondents to the questionnaire were not queried about their motivation in accepting offers of new positions. It may be assumed that these were responses to offers of higher pay, of opportunity to engage in independent research or research under more desirable conditions, or to locate in geographical areas attractive to the families of the scientists concerned.
- PREVIOUS EDUCATION OF WORKING LIFE SCIENTISTS
In the foregoing summary, the initial training of working life scientists was categorized in disciplinary terms that are familiar as the titles of academic departments and that are employed in most statistical collections. However, the reader who has considered earlier chapters will have recognized that these conventional subdisciplinary titles have, in considerable measure, lost their meaning and convey false distinctions. Whereas biochemists were formerly concerned largely with elucidation of metabolic maps, they may today be concerned with macromolecular structure, the chemistry of cell-cell recognition, or the phenomena responsible for atherosclerosis. Not so long ago, microbiologists were overwhelmingly concerned with the taxonomy of microbiological forms, yet today they may be concerned with genetic mechanisms or the nature of the immune response to invasion by some specific organism. Hematologists, who only yesterday were describing changes in the morphology of blood cells in leukemia as seen with a light microscope, are now intimately involved in understanding the manner in which nucleic acids control the differentiation process among white blood cell types. Physiologists, who formerly engaged in studies of the mechanics of muscular contraction or morphological changes induced by steroid hormones, are today inquiring into mechanisms of transmembranal transport or the molecular events by which steroid hormones affect protein biosynthesis in receptor cells. Botanists, once engaged in taxonomic studies or in gross plant physiology, are today concerned with the phenomena by which plants interact with other organisms and with their environment, the cardinal aspects of ecology, while zoologists may be concerned with all those aspects of the environment that have favored rapid proliferation of new species in one set of circumstances or remarkably prolonged survival, unchanged, of other species, studies that embrace all aspects of ecology, genetics, biochemistry, and physiology. Even more dramatic have been the changes in the character of research in clinical medicine, pathology, and pharmacology. Investigators in these areas have learned to use the most recent developments in understanding such phenomena as protein structure, enzyme kinetics, transmembranal transport, neural transmission, immunochemistry, viral reproduction, lipid metabolism, and behavioral genetics as they explore disease mechanisms in man or animals, design and test new drugs, or prepare a patient for organ transplantation. And their laboratories cannot be distinguished from those of other scientists so engaged.
Because of these rapidly evolving and profound trends, it appeared desirable to reconsider individual scientists, not under classical disciplinary labels, but in relation to the nature of the research conducted during their initial formal education in graduate school and in relation to the research in which they are currently engaged. That two individuals are studying cellular structure and function is more significant than that one considers himself a zoologist and the other a botanist. The plant pathologist may have more in common with an animal pathologist than with a plant taxonomist, and similar considerations are obvious for plant and animal physiologists, or for plant, animal, and microbial geneticists, for example.
Thus, we have found it useful to recategorize life sciences research into the following dozen classifications:
Behavioral biology
Cell biology
Developmental biology
Disease mechanisms
Evolution and systematic biology
Molecular biology and biochemistry
Pharmacology
It will be evident that even these categories are somewhat arbitrary and are by no means mutually exclusive. They fail to make clear the fact that biochemistry, a research area itself, is also the common language and the tool for almost every other entry in the classification scheme. However, the questions being asked of nature by scientists within each category are sufficiently distinct to permit self-identification by our respondents, while providing a more revealing description of the life sciences endeavor than that offered by more traditional disciplinary titles.
Tables 9 and 10 summarize the current research areas of some of our respondents, comparing their current areas of involvement with the disciplines and research areas in which they had been trained as graduate students. As a consequence of an awkwardness in the design of the layout of the printed questionnaire, almost a quarter of all respondents failed to provide information concerning the research fields, as here categorized, in which they had been trained and in which they are currently engaged. However, as indicated in Appendix A , it appears fair to assume that the patterns revealed by those who did not overlook this question are representative of the total.
Comparison of Current Research Areas with Areas of Most Recent Ph.D. or D.Sc. Degree.
Comparison of Current Research Area with Disciplines in Which Life Scientists Were Trained.
As indicated by the diagonal of Table 9 , current research in any given area is conducted predominantly by individuals who were trained in that area, varying from 49 percent of those currently engaged in behavioral biology to 85 percent of those working in genetics. Equally impressive, however, is the degree of intellectual migration among research fields. Thus, 48 percent of all those trained in morphology are now engaged in some other area, as are 39 percent of those originally trained in cell biology, 33 percent of those trained in developmental biology, and 30 percent of those trained in physiology. Maximum field retention was found among those trained in pharmacology, ecology, genetics, and molecular biology and biochemistry. Perhaps the most striking fact shown by the table is that every possible crossover was reported. Noteworthy, too, are the fields that, on balance, have either attracted more investigators than they have lost, or vice versa. The “gainers” include molecular biology and biochemistry, behavioral biology, cellular biology, disease mechanisms, ecology, and pharmacology. The most significant “losers,” in absolute numbers rather than percentages, were genetics, morphology, nutrition, and physiology, with developmental biology and systematic biology remaining approximately in balance.
Many biologists currently consider that there has been a rapid growth in the opportunities for fruitful studies in behavioral and developmental biology and in ecology. But these data indicate that, although there has been some modest influx into these fields, it is not yet particularly striking, although graduate enrollments have been affected in the predicted directions. Moreover, the changes are generally immediately lateral in the sense that most of those who have changed research areas have moved into areas in which they can apply the skills and insights of their primary training. This is most certainly the case for the 184 of 287 individuals who left molecular biology and biochemistry to enter upon studies in cellular biology, disease mechanisms, pharmacology, or physiology, as it must also be true for the 317 individuals who left physiology to enter other biological categories.
Only 741 scientists were sufficiently certain of their plans to change research areas in the future to so indicate. And again, the planned changes were, in the main, relatively conservative ( Table 11 ) and into closely related areas, e.g., molecular biology to genetics, genetics to molecular biology, physiology to pharmacology, botany to ecology. Molecular biology will be the chief gainer (19 percent of all who plan to change), largely from cellular biology and physiology. However, it will lose a slightly larger number (20 percent), mainly to cell biology, developmental biology, and disease mechanisms. Disease mechanisms attracts the second largest group (15 percent), largely from among those now engaged in cellular biology, biochemistry, and physiology, while developmental biology also seems attractive to those in the same group of research areas (12 percent). The survey revealed a particularly interesting trend. Some ecologists indicated plans to enter behavioral biology, while a significant number of physiologists and students of disease were seriously considering switching to ecology.
Projected Research Areas of Some Life Scientists.
Moreover, the perhaps not unexpected conservative migratory pattern is again evident from the responses of life scientists who intended to change the biological material with which they were working. In a general way, those now seriously contemplating such a change are, in the main, thinking of switching either to the next higher or the next lower level of biological organization, e.g., from broken cell preparations to cells or tissue culture or to molecular systems; or from intact organs to either intact organisms or cellular preparations.
Table 12 relates research areas to the principal employers of the 8,139 individuals for whom such information is available. Of this subset, institutions of higher learning employed 68 percent, the federal government 14 percent, industry 9 percent, and all other nonprofit organizations, hospitals, etc., 9 percent. Noteworthy are the high levels of employment by the federal government of those studying ecology and disease mechanisms; the government shows much less interest in developmental biology, morphology, and pharmacology. Private business employs an unusually high fraction of all nutritionists and pharmacologists, but appears to have little interest in ecology, systematic biology, or morphology.
Distribution of Investigators in Various Research Areas by Principal Employer.
A small insight into the changing dynamics of the life sciences is provided by observation of the fraction of the total population within each research area under 34 years of age. This fraction is remarkably close to 21 percent for virtually all research areas, with a few interesting exceptions. Only 11 percent of those engaged in the study of disease mechanisms are within this age group, presumably reflecting the long period of residency training for physicians. In contrast, 23 percent of those in developmental biology and 28 percent of those in molecular biology and biochemistry were under the age of 34 at the time of this survey, indicating that in the recent past these two fields, as compared with the other research areas, have become increasingly attractive to young scientists. Only 18 percent of all those attracted into the life sciences from the physical sciences were within this age group, indicating that there has been no dramatic upsurge of interest in the life sciences among young chemists or physicists.
The reverse situation is in accord with the same suggestions. For the entire population, 18 percent were 50 years of age or older, but only 12 percent of those in molecular biology and biochemistry fell within that age range, in contrast with 25–28 percent in the areas of disease mechanisms, evolutionary and systematic biology, morphology, and nutrition.
Of some interest are the attributes of the group of investigators originally trained only as M.D.'s or in the other health professions. They are older, with only 15 percent under 34 years of age, but 42 percent within the age span 40–49. Logically, disease mechanisms constitute their principal single interest (27 percent of the total), but they are also represented in every other research area with the exception of systematic biology, major interests being physiology (22 percent), molecular biology and biochemistry (15 percent), cellular biology (9 percent), and pharmacology (8 percent).
The 456 women showed only a few distinct tendencies to differ from the distribution of the men. Women tended to avoid physiology, ecology, and systematic and behavioral biology, and 28 percent of all female respondents work in molecular biology and biochemistry.
- POSTDOCTORAL TRAINING
Prior to World War II, postdoctoral research training experience was a privilege granted very few young scientists. Fellowships were scarce, and only the most highly talented could aspire to such opportunity. Since available research grants were decidedly limited in size, few senior academic investigators commanded the means to support eligible new M.D.'s or Ph.D.'s desirous of embarking upon the apprentice training characteristic of the postdoctoral experience. That situation no longer obtains. Postdoctoral experience has become almost the norm rather than the exception, and we are entirely convinced that this is in the national interest.
However, the situation has given rise to concern among those less closely associated with research in these disciplines. For example, agencies that provide support for postdoctoral training are uncertain of its value. Educational institutions in which postdoctoral fellows abound are uncertain of their institutional responsibility for this enterprise. Institutions that, perhaps until 1969, have had difficulty in recruiting sufficient staff to meet teaching obligations—largely the four-year colleges and junior colleges, but also a significant number of medical schools, as well as industry and some federal laboratories—have complained that the postdoctoral system is a holdup in the pipeline that, in the steady state, keeps a substantial number of bright young investigators out of the regular job market. We appreciate these problems, but consider that the benefits of postdoctoral education far outweigh these transient difficulties. Let us consider here the postdoctoral training experience of our responding population of life scientists. In the following chapter there is a summary of the numbers and activities of postdoctoral fellows in training in 1967–1968, as well as an analysis of the contribution of postdoctoral education to the operation of the entire endeavor.
Of the 12,151 investigators in the study, 5,041 had had at least one postdoctoral appointment, including 1,402 M.D.'s who had had postdoctoral experience in which research was their major responsibility. Three fourths of those who had had postdoctoral experience are now in academic life. Indeed, 45 percent of the 8,143 scientists now employed by universities had enjoyed postdoctoral experience, compared with 21 percent of the scientists in industry and 31 percent of those in the federal establishment. Taken across all disciplines, postdoctoral experience somewhat enhances the opportunity for employment in the federal government and markedly enhances the opportunity for employment in the universities. It is our impression that in universities with major commitments to graduate education and research, measured in supporting dollars and number of graduate students, faculty appointments for individuals who have not had postdoctoral experience are probably rare indeed. According to a National Academy of Sciences study of postdoctorals, * 74 percent of all new appointees to the rank of instructor or assistant professor in 21 departments of biological sciences in 10 “leading” institutions either came from other university faculties or had just held postdoctoral appointments.
However, the trend to postdoctoral education is not universal across all biological fields. For example, of the 855 individuals with graduate training in agricultural fields, only 35 had had postdoctoral appointments. In contrast, postdoctoral training was commonplace among M.D.'s since it has become the conventional medium for obtaining research training among this group.
As shown in Table 13 , postdoctoral training was less frequent among botanists (29 percent) than among biochemists (53 percent), with the other disciplines ranging in between. Postdoctoral training was frequently taken in fields other than those in which scholars had their initial doctoral experience. Thus, of the zoologists and botanists who did take postdoctoral training, less than half did so in zoology and botany departments. Again, the biochemists appear as the other extreme. Not only did a larger fraction of biochemists than other life scientists take postdoctoral training, but a decidedly larger fraction remained within biochemistry for their postdoctoral experiences. Since an additional 540 individuals who had taken their original graduate education in fields other than biochemistry sought postdoctoral training in biochemistry, postdoctoral education is a major aspect of life in biochemistry departments. Large numbers of those trained in biochemistry in graduate school later work in other disciplinary areas, while many individuals enrich their original disciplinary education by a one- or two-year postdoctoral experience in biochemistry and then, when they become independent investigators, return to their original disciplines and research areas or enter yet other research areas.
Postdoctoral Experience of Scientists in a Limited Group of Biological Disciplines.
These data uphold one of the primary arguments in support of the trend toward postdoctoral experience as a normal component of the education of those who later will espouse careers in which research is a major activity, viz., that this constitutes a unique opportunity to broaden one's horizons, learn new techniques, and become familiar with the style of other subdisciplines, while profiting by the examples of different master scientists. The overall situation is reflected in the totals of Table 13 . Of 5,765 Ph.D.'s in this file, 2,395 undertook postdoctoral experience, of whom 1,463, or 61 percent, extended their experience in the same disciplines in which they had studied in graduate school. But the impression that postdoctoral experience is a continuation of graduate education in 61 percent of all cases is misleading, since it is weighted by the fact that more than half of all of those who did experience this continuation were biochemists. If the biochemists are excluded, only 50 percent of the remaining scientists who undertook postdoctoral training did so in their graduate disciplines. Moreover, such an experience is but rarely a mere continuation of graduate education. This is borne out by the following consideration: In a subfile of 3,234 postdoctoral fellows, only 14 percent had taken postdoctoral education in the same university in which they had obtained their doctoral degrees, and only 6 percent in the same departments that had awarded their doctoral degrees. This migratory pattern is particularly evident among the M.D. population. However, about one third of all Ph.D.'s in agriculture and forestry who undertook their postdoctoral training—a rather small group—did so in their original universities and, indeed, in the departments that had awarded their degrees. The rather small proportion of students who remained in the same department for postdoctoral study was almost twice as great in public universities as in private universities.
In sum, it is clear that the norm for postdoctoral experience, by a wide measure, consists of apprenticeship to a different set of investigators in an environment different from that in which graduate education has been completed. Further, in the experience of our panelists, the current internal heterogeneity of the classical disciplines assured that even the postdoctoral trainee who remains within his original discipline is likely to engage in a problem remote from his graduate research experience. The biochemist who studied intermediary metabolism may later become preoccupied with the mechanism of enzyme action; the physiologist who traced neural pathways as a graduate student may focus upon ion transport across the nerve membrane during his postdoctoral years. The botanist who was concerned with nutritional requirements for plant growth may later become involved in the ecology of a cornfield, while the entomologist concerned with patterns of insect distribution may switch to a study of insect sex attractants. Intellectual inbreeding is rare in the life sciences community, and the postdoctoral experience is among the chief means of assuring the hybrid vigor of the entire enterprise.
A few notes comparing the bioscience subculture with the subcultures of the physical and social sciences may be warranted. The data in support of the following statements are derived largely from the recent National Research Council study of postdoctoral education, The Invisible University . *
In the nation's leading academic institutions, postdoctoral experience has become the expected prelude to faculty appointment. In recent times, 70 to 80 percent of all initial faculty appointments at such institutions in physics, in chemistry, in biology departments of faculties of arts and sciences, and in the preclinical departments of medical schools have been made to individuals with postdoctoral experience either at the same or at some other institution. In contrast, initial faculty appointments in the social sciences, the humanities, and engineering relatively rarely require postdoctoral experience. The play of the academic marketplace is such that the frequency of postdoctoral experience among initial appointees to the faculty decreases with the general academic status of the institution. Postdoctoral experience is less frequent among the faculties of “developing” universities, is rare for scientists who are appointed to the faculties of liberal arts colleges, and is even less common among those who enter industry.
The converse is equally evident; 30 to 40 percent of all relatively young faculty at all universities who have not had postdoctoral experience feel this lack in their current professional lives. In all branches of natural science, promotion up the academic ladder occurs somewhat less rapidly for those who have not had postdoctoral experience, although this may reflect similar appraisal of human potential by the committees who select postdoctoral-fellowship recipients and those who recommend academic promotions, rather than the intellectual rewards of postdoctoral study. These trends are undoubtedly enhanced by the advice given to aspiring scientists by their mentors in graduate school, who strongly urge students in the natural sciences to undertake postdoctoral experience if they aspire to academic careers but rarely do so when this is not the case. In general, such mentors recommend a postdoctoral experience of about two years, with a specific senior scientist in a field somewhat different from that in which the student's dissertation research was conducted, thereby broadening his understanding of his discipline. When queried, postdoctoral students advance the same general purpose as their reason for undertaking postdoctoral study, but place more emphasis than do their graduate mentors upon the acquisition of additional research techniques.
Attempts by statistical means to assess the influence on subsequent scientific productivity of postdoctoral training are not revealing. Differences among those who took postdoctoral training immediately after graduate school, those who deferred such training for several years, and those who had no such training are trivial when measured by counting numbers of scientific publications, reviews, books written, and similar measures. What cannot be assessed by this means is the quality of the work or its significance to the field. One indicator has been reported in The Invisible University * : the fact that papers published by those who have had postdoctoral experience are cited about twice as frequently in the Citation Index † as are papers by those who have not had such experience. Statistically, frequency of citation of a paper is some measure of its significance or fundamentally. It is our contention that, in all scientific fields, scientific boldness—willingness to venture beyond the frontier or to undertake large and challenging problems—is established relatively early. Certainly, if this is not encouraged in graduate school or in the immediate postdoctoral years, it is rarely evident in subsequent careers. But statistical assessment of this all-important quality is not readily feasible; hence, the enhanced opportunity to develop such habits of mind is another argument that we would advance in support of a year or two of postdoctoral study, preferably not in the same institution or with the same mentor that provided the graduate experience.
Data purporting to compare the consequences of graduate or postdoctoral study in the 10 or 20 leading academic institutions with those in other institutions are probably not completely valid. The selection process that operates at the level of admission to graduate school and then to postdoctoral study in the most productive academic laboratories already serves as a screen almost sufficient to assure the ultimate outcome. It is not readily possible to distinguish between the consequences of differences in the quality of the educational experiences in such institutions and the consequences of the quality of the initial human input. Certainly it must be undeniable that those most highly qualified will benefit most from a stimulating environment in which science is being conducted at its outermost frontiers.
- EDUCATIONAL LIMITATIONS
An attempt was made to estimate the extent to which working life scientists sense deficits in the educational preparation for their careers. Respondents to the questionnaire were asked to state whether their current research programs are significantly limited by their own educational preparation in chemistry, mathematics, physics, electronics, statistics, other areas of the life sciences, or the use of computers. In all, 4,396 scientists, 30.6 percent of the entire responding population, indicated that full development of their current research effort is indeed very seriously hindered by insufficient personal training in one or more of these disciplines. Lack of knowledge of chemistry was most frequently felt to be limiting (1,766 individuals), followed by computer science (1,569), mathematics (1,427), statistics (1,136), other biological sciences (1,085), and electronics (983), with only 498 life scientists acutely aware of insufficient personal training in physics.
Scientists in academic institutions were not distinguished from those working in nonacademic institutions with respect to this pattern of perceived inadequacies, although 38 percent of academic personnel were aware of some such limitation, and only 30 percent of nonacademic scientists were. In both groups, those in the middle of the age range (35–50 years) were about 30 percent more likely to be aware of such deficits than were younger or older investigators. Again, however, age was essentially without influence on the pattern of perceived disciplinary insufficiency; the rank order of disciplines cited above for the entire population was characteristic of the youngest, oldest, and midrange investigators alike.
- WITH WHAT MATERIALS DO LIFE SCIENTISTS WORK?
The panorama of the biological universe offers such remarkable and diverse organisms, ecological situations, environmental responses, and unanswered questions at levels varying from the molecular to the cosmic that it is not surprising that research biologists employ an almost equally disparate and diverse variety of approaches to the questions they put to nature. In Table 14 is displayed a representation of primary research materials and the extent to which these are utilized by those who work in various biological research areas.
The Research Materials of Life Scientists.
It may come as a surprise to some that mathematical models are utilized by representatives of almost every research area, most frequently by those engaged in the study of physiology, molecular biology and biochemistry, genetics, or biophysics and, increasingly, in studies of ecology. Molecular models are to be found in virtually every biochemical laboratory, and the refined, precise models now available have become an extremely important tool for those seeking to relate molecular structure to biological function. Indeed, 46 individuals stated that such models constitute their primary materials.
It was somewhat surprising to find 6 percent of the entire surveyed population engaged primarily in the development of analytical procedures of various types. Study of molecular systems, utilizing highly purified materials of natural origin, engaged 10 percent of the total population, including one third of the biochemists. A somewhat greater proportion of life scientists were studying the behavior of subcellular organelles, isolated or in situ . Such materials are utilized by scientists, except the ecologists, in all research areas and, as one might expect, are a principal preoccupation of cell biologists and biochemists. A small proportion (3 percent) of our population, most notably the cell biologists, were learning to use disassociated preparations of living cells, from either plant or animal sources, as primary tools in their studies. Tissue culture was twice as popular and was utilized by at least some scientists, including behavioral biologists, in every research area, while intact tissues and organs claimed the attention of 12 percent of the total population, involving all research categories except ecology—most notably morphologists, pharmacologists, physiologists, and developmental biologists.
Intact individual organisms were the test objects of one third of all life scientists in the study, notably the behavioral biologists and those studying disease mechanisms, ecology, systematic biology, genetics, nutrition, pharmacology, and physiology. Decidedly smaller numbers of scientists addressed themselves to entire populations of organisms or to ecosystems.
Of interest is the fact that the pattern of use of materials by those with original training in the health professions cannot be distinguished from that of the remainder of the population; their primary research materials simply reflect the pattern of all others in the research areas in which they now engage. Accordingly, their major research materials are whole organisms (32 percent), tissue and organ systems (23 percent), subcellular fractions (13 percent), cell cultures (8 percent), and molecular systems (9 percent).
Within each research area a few individuals are engaged in comparative studies either within a single phylum or plant division or across several phyla or plant divisions. Although students of evolution and systematic biology were the most numerous such group, these were only 44 of the 123 individuals so engaged.
- WITH WHAT SPECIES DO LIFE SCIENTISTS WORK?
The diversity of living nature never fails to astonish. The workings of evolution have resulted in millions of distinct species of living forms, unicellular, plant, and animal, all located in the thin web of life, which is a film on the surface of our planet. These are the objects of study for life scientists. But which species should one study? The answer depends upon the question that has been raised. Some species are of interest because they are the basis of our agricultural economy. Some make the world more beautiful and exciting; some cause disease of man, plant, or animal. Sometimes even the most obscure species provide excellent models for study of complex biological phenomena. And surely a proper object for study by man is man himself! Thus there are valid reasons for the study of a great variety of species.
Some species are of interest because they are intermediate links in a food chain, because they survive under what appear to be improbable conditions, or because they represent evolutionary extremes. Still others are of interest because they offer unique opportunities to study phenomena of general importance but difficult to analyze or observe in more common species. For example, the nerve net of the crab is of interest as a prototype of the more complex nervous system of the mammal; the response of certain insects to sugars can serve as a model for some aspects of the physiological bases of behavior; the “alarm reaction” of the clam is highly instructive with respect to certain reflex activities; the photosynthetic properties of the chromatophores of purple bacteria and of certain algae are more readily studied than is photosynthesis in a higher plant; regulation of the genome of a bacterium serves as a model for the process of differentiation in a higher organism; and the giant axon of the squid is the favorite test object of numerous neurophysiologists. Nutritionists long since seized on the omnivorous white rat as a model for human nutritional requirements, but primates may be more instructive with respect to human behavior or reaction to disease. The pig offers a surface area and mass somewhat comparable to that of man, and thus should serve as a model for human response to radiation. Comparison of the properties of hemoglobins from a wide variety of species elucidates those properties of the hemoglobin molecule that are imperative to its physiological function, and frog muscle has taught us much of what we understand of muscle physiology and its molecular aspects. The list is well-nigh endless.
And so it is that life scientists continue to study or exploit the properties of a great diversity of organisms. In a highly compressed form, this is displayed in Table 15 . Each of the respondents to the questionnaire was given a choice of 58 genera, phyla, or larger divisions of the plant, animal, and microbial kingdoms and was asked to indicate no more than two that most closely described the objects of his study. Hence, the number of specific responses exceeded the number of respondents. But hundreds of investigators indicated that necessarily and properly they should indicate more than two such entries.
Biological Materials Studied by Life Scientists.
Perhaps the aggregated totals are of greatest interest: 21 percent of all scientists dealt with one or another micro-organism, 15 percent with plant forms, and 54 percent with animal forms. None of the categories of living forms was totally ignored by the current activities of life scientists but, clearly, some are more attractive than others. Viruses and bacteria are the concern of scientists in each research area, particularly those who study disease mechanisms, cell biology, and molecular biology and biochemistry. Lower plants engage the attention of all but the nutritionists and pharmacologists, while higher plants attract the attention of all but the pharmacologists. Invertebrates are of great interest to the ecologists and the systematists as well as to the behavioral biologists, who see in them models for the behavior of more advanced forms. Surprisingly little attention is being given to the species of fish that dominate our commercial harvests, whereas other fish, amphibia, reptiles, and birds are receiving greater attention. Of the mammalia, man and the common laboratory rodents are the most frequent study objects. The great utility of the latter is indicated by the fact that, whereas ecologists and systematic biologists pay them scant heed and only 6 percent of all geneticists make use of their particular attributes, these species are utilized by 12 percent of the behavioral biologists and 37 percent of the pharmacologists. Domestic mammals, i.e., cats and dogs, are particularly useful to the physiologists, pharmacologists, nutritionists, and morphologists and are used to some degree by almost all other groups.
Although 5 percent of all behavioral biologists and 4 percent of the morphologists report that they work with small primates, primates are little used by workers in other scientific areas. However, there is reason to think that this reflects not the utility of these species, but the great costs involved in their acquisition and maintenance, which have inhibited, if not prohibited, their utilization for a variety of studies in which they could be extraordinarily useful.
In contrast, millions of species currently go unstudied, and many others are under scrutiny by only one or two investigators. When, from time to time, such an investigator directs attention to some unique or remarkable attribute of a seemingly esoteric species, it can rapidly claim the attention of many other scientists, an incident that has recurred many times in the past. Thus, the bacterium Escherichia coli has become the most thoroughly studied of all cells, while both neurophysiologists and molecular biologists have recently seized upon the tiny marine organism Aplysia because of its easily studied giant nerve cells. In any case, the diversity of species under study demands an equal diversity of laboratory accommodations for their culture or maintenance. This may engender substantial expenditures and contribute much to the cost of scientific investigation, particularly in extreme instances. Elaborate facilities are required for the conduct of research employing cells in culture. Inadequate accommodations, overcrowding, or infestation can render a colony of dogs or rodents useless to the investigator and give rise to misleading data. Humane considerations demand that larger domestic mammals—cats, dogs, and primates—be housed in decent quarters, be wellnourished, and be subjected to the minimum of trauma commensurate with the purposes of study. This in turn creates further serious financial requirements, which should be borne by some institutional mechanism and not met by taking funds from personal research grants made to individual investigators. Certain plants and animals require carefully controlled environments; a continuing supply of virus may require a colony of host animals, a large-scale fermentor, or a large tissue-culture facility. Most importantly, all these demand substantial expenditures merely to assure a supply of the biological entity to be studied before the research proper can be undertaken.
- WHAT FACILITIES AND TOOLS DO LIFE SCIENTISTS USE?
The classic image of the biologist is an aging gentleman, wrapped in a dirty laboratory apron, in a musty laboratory surrounded by museum jars, an ancient, battered microscope, staining jars for microscope slides, and perhaps an unwashed dissecting table. If that image ever corresponded to reality, it no longer does. As the questions we ask of nature become more sophisticated and the information we seek becomes more remote from that which we can acquire with our naked senses, the requirements for the conduct of research in the life sciences become more complex. Today, in order to achieve his ends, the investigator may have to travel thousands of miles from his home base, armed with telemetering equipment, tape recorders, or remote sensors. He may require a floating laboratory, a deep-submersible vessel, a reconnaissance plane, or even a satellite equipped with infrared sensors. He may utilize the gadgetry of modern biochemistry— ultracentrifuges, equipment for optically following the course of kinetic processes on the scale of milliseconds or of molecular-relaxation times (10 −9 sec), for the quantitation of visible or ultraviolet light or radioactivity. His laboratory may be what amounts to a small electronics plant equipped with the complex electronic apparatus needed for the study of neurophysiology, and his experiment may be guided by an on-line computer. Increasingly, the tools of any biological subdiscipline tend to become the tools in many other areas of biology. As we have noted repeatedly, this is particularly true of the tools of the biochemist, which have become the tools of all biologists.
Specialized Biological Research Facilities
Table 16 summarizes the replies from respondents whose completed questionnaires usefully indicated their utilization of specialized research facilities. The spectrum of such activity is broad indeed. For example, we were surprised at the high rate of utilization of controlled field areas, which seemingly are employed by participants in each of the research areas. Computer centers are available to and utilized by a strikingly high fraction of all life scientists, and general animal care facilities appear to be utilized by almost half the scientists covered by our survey. Indeed, it is difficult to correlate specific types of facilities with specific research areas. Notable exceptions include the 87 percent of all systematists and 44 percent of ecologists who utilized taxonomic research collections, the 51 percent of cell biologists who employed cell- or tissue-culture facilities, and the 76 percent of all pharmacologists who made use of general animal care facilities. The existence of the specialized facilities listed here was known to the Survey Committee, but the extent of use was not anticipated.
Utilization of Specialized Biological Research Facilities.
Rarely can the cost of acquisition and maintenance of such facilities be justified by the research program of a single investigator; hence, no small or medium-sized institution can hope to have a complete selection of these opportunities for conduct of research. This has the effect of either limiting the capabilities of the staff of such institutions or so affecting their recruitment patterns that, at each institution, there are clusters of investigators whose research requires easy access to the same major research facility. For smaller institutions, this fact, in turn, may well prevent the assembly of a staff broadly representative of biology.
Major Instruments
Table 17 displays the utilization of major instruments by life scientists during 1966–1967. Like Table 16 , this table is limited to those respondents whose replies to the questionnaire were found adequate to the purpose. And, as in Table 16 , what is impressive is the extent of use of the wide variety of instruments listed and the relative amount of use without regard to specific research areas, again with a few notable exceptions. This table well illustrates how the tools developed for biochemical studies have become the tools of biology in general; this is evident in the use pattern of centrifuges, gas chromatographs, amino acid analyzers, scintillation counters, infrared and ultraviolet spectrophotometers, as well as electrophoresis apparatus. These common tools of the biochemical laboratory are now the common tools of the biological laboratory. Specialized uses of instruments will, however, be found in the table. For example, large-scale fermentors are used largely by biochemists; multichannel recorders are required by physiologists and pharmacologists; small special computers by physiologists. Biochemists are pioneering in the use of ultrasonic probes, and electron paramagnetic resonance and nuclear magnetic resonance spectrometers, as well as instruments for measuring circular dichroism. The physiologists are the major users of infrared carbon dioxide analyzers, and the clinicians interested in disease mechanisms utilize complex electronic systems for monitoring human physiology, while systematists use telemetry and sensitive tape recorders.
Utilization of Instruments by Life Scientists.
The utilization of the electron microscope is particularly revealing. This instrument, slowly introduced into biological laboratories in the years following World War II, is now used by investigators in every research area. In absolute numbers, those interested in molecular biology and biochemistry, cellular biology, disease mechanisms, and physiology are the principal users. But 48 percent of all those studying morphology and 44 percent of those studying cellular biology made use of this instrument. The great expense of acquisition and maintenance of these instruments prevents the figures for utilization from approximating 100 percent of those in both of the latter research areas.
One should not leave the subject of instruments without a tribute to the instrument-manufacturing industry. This highly competitive industry has frequently been a jump ahead of most life scientists. In general, instrument manufacturers have recognized needs and potential uses before the scientific community has. Yet, as each instrument has become available—e.g., ultraviolet spectrophotometers, electrophoresis apparatus, scintillation counters, electron microscopes, and multichannel recorders—not long thereafter the scientists involved have wondered how they had ever made progress before these commercial instruments became available. As the markets grow, the instruments become more refined, more reliable, and more versatile, thereby enormously enhancing the reliability, sophistication, and ease of performance of biological research. The availability of such instruments has been made possible by the very scale of federal support of the life sciences. By creating a sufficient market, the manufacturer has, in turn, been able to achieve economies of large-scale production, keeping the unit cost and sales price down. (It is ironic that, although the electron microscope was developed by an American firm, and this country is the major market for this instrument, no American manufacturer now supplies it.)
Nor should we fail to acknowledge our debt to our brethren in physics, chemistry, and engineering. From them came the electron microscope, spectrophotometers, the electron paramagnetic and nuclear magnetic resonance spectrometers, ultrasonic gear, the great variety of oscilloscopes, x-ray crystallographic analysis systems, the laser, telemetry, and a host of other devices. To their designers and developers, the biological community extends its gratitude.
- THE RESEARCH GROUP
Research in the life sciences is “small science”; only rarely is it organized around some very large and expensive piece of apparatus or facility. Whereas much research in other areas of science revolves about large accelerators, research vessels, telescopes, balloon-launching facilities, rocket facilities, or large magnets, for example, there are few parallels in the life sciences. Occasional exceptions include relatively elaborate hyperbaric facilities, primate colonies, colonies of germ-free animals, phytotrons or biotrons, biosatellites, museums, or marine-biology stations. But these are the exceptions rather than the rule, and even in these instances, the facilities in question are actually utilized by numbers of small research groups, each pursuing its own questions in its own way, while taking advantage of the availability of the facilities. In very few instances have the various groups that, collectively, used such a facility comprised a coordinated whole with common goals and objectives. The functional unit of research in the life sciences, therefore, usually consists of a principal investigator and the postdoctoral fellows, graduate students, and technicians who work with him. According to data collected by the Study of Postdoctoral Education of the National Academy of Sciences, * the mean such research group, in addition to the faculty member, is 6.1 members in academic biology departments, 7.6 in biochemistry departments, 5.3 in physiology departments, and 4.0 in clinical specialties. These may be compared with 5.8 members in physics and 8.3 in chemistry. When, however, research groups without postdoctoral are considered, these units are distinctly smaller, receding to 4.6, 3.9, and 4.0 in biology, biochemistry, and physiology, respectively, and 3.2 and 5.2 in physics and chemistry.
This scale of operation was borne out by reports from the individual investigators surveyed in the study. For all principal investigators, the mean was 6.5 persons per research group, in addition to the principal investigator himself, ranging from 4.4 for investigators engaged in studies of systematic biology to 8.0 for those studying disease mechanisms. Perhaps surprisingly, the sizes of groups were much the same in academic and nonacademic laboratories. Approximately equal numbers of co-investigators and professional staff are found in both classes of laboratories. The graduate students, who vary in academic laboratories from 1.5 to 4.0 students per group (the extremes being represented by morphology and behavioral biology, respectively), with an overall average for all biological disciplines of 2.2 students per group, are replaced in nonacademic laboratories by technicians and other supporting staff.
Thus, in general, the typical academic laboratory contains a principal investigator, a co-investigator, and one other scientist with a doctoral degree who may be a visiting scientist, postdoctoral fellow, or continuing research associate, two technicians, and two or three graduate students. Federal laboratories may have one or two postdoctorals in place of the graduate students, while industrial laboratories utilize additional technicians. The routine tasks of the laboratory are generally performed by the technicians, while the graduate students and postdoctoral fellows serve as junior co-investigators and colleagues for the principal investigator. In our view, such a research group does indeed constitute something close to optimal for the conduct of “small science,” particularly in the life sciences. Graduate students and postdoctorals are spared some of the drudgery of routine analyses after they have learned to perform such analyses and understand their limitations, and the total group combines a mixture of experience, expertise, ideas from other disciplines, and youthful enthusiasm. We can only conclude that, however haphazard the various mechanisms by which such an enterprise is funded, the average working unit is sufficiently large to attain an intellectual critical mass and to sustain the pace of exciting investigation while training the novice investigator for his future career.
Although this report gives emphasis to the research and education endeavor of the universities, it remains possible for dedicated scholars to pursue meaningful research in the biology departments of the independent four-year colleges. Biology is still mainly “small science,” and research in many subdisciplines can be conducted with relatively modest support. When access to major equipment is required, this is frequently arranged with the faculty of a nearby university or undertaken during the summer at some properly equipped institution. These efforts constitute a significant part of the total life sciences research endeavor.
There are, however, important exceptions to this “small science” pattern. Decidedly larger aggregates of scientists, focused on a single goal, have been brought together to design a biological experiment for a space probe or to study the ecology of a major biome. The integrated approach to environmental research, stimulated by the International Biological Program, promises to open new levels of understanding of the functioning, resilience, and critical sensitivities of man-dominated ecosystems. In this program, teams of ecologists, social scientists, and physical scientists— as many as 150 individuals—cooperate in the analysis of entire ecosystems, such as the Western grasslands, the Eastern deciduous forests, or the Southwestern desert. Their data are compiled, coordinated, and utilized to construct mathematical models of these large systems, one day to be integrated with models of the atmospheres of the same regions. These systems involve so many components and multiple interactions that realistic abstractions or simplifications must be designed for simulation on large digital computers. The model is a combination of mathematical expressions and statistical probability distributions representing the processes and interactions of the system, as from soil to plant or plant to animal, and the impact of temperature on energy flow. A properly designed model can be used to suggest the potentially most fruitful field experiments from among the multitude that might be conducted, to identify gaps in existing knowledge through deficiencies in model performance, and to suggest optimal courses of action in managing real-world ecosystems. In the medical schools, large groups with representatives from several clinical or preclinical departments coalesce to collaborate on some aspect of cardiovascular, neurological, or neoplastic disease. These groups can number from 20 to 200 scientists and may well serve as forerunners of an era of “big biology.”
- WHAT DO LIFE SCIENTISTS DO?
The average life scientist employed in an institution of higher learning devotes about half his time to research, 10 to 20 percent to administration, a fourth to a third of his time to instruction, and the balance to assorted other responsibilities. The actual distribution, of course, varies with the type of institution and the specific disciplinary field and according to whether he has clinical responsibility. This pattern is clearly in contrast with that of life scientists employed by nonacademic institutions, for whom research is, to an even greater extent, their dominant responsibility, demanding about 70 percent of their effort, while the remainder of their time is largely devoted to administrative responsibilities. Surprisingly, nonacademic scientists report that they engage in instruction that varies in percentages of their time from 0 to 10 percent—about 3 percent for the entire group but 8.5 percent for physicians. The physicians also give a sixth of their time to clinical care and hence can devote only about half their time to research. Some pertinent data in this regard are summarized in Table 18 .
Percentage Distribution of Work Time of Some Life Scientists.
The same set of respondents, 6,125 scientists in academic institutions and 3,054 scientists in nonacademic institutions, were also queried with respect to whether the research in which they were engaged was basic, clinical, or applied. It was made clear that these designations were not necessarily mutually exclusive and, indeed, that an individual could check more than one of these categories if he felt that this was appropriate, particularly if he was engaged in more than one research project. Some of the resultant data are shown in Table 19 . It is not surprising that scientists outside the academic world engage in applied and clinical research. But it may be surprising that 22 percent of all life scientists in institutions of higher learning indicated that their research is applied in some degree. By their own judgment, 76 percent of academically employed physicians indicate that they are engaged in basic research, and only 12 percent state that the research that they are doing is “applied” in some fashion. Quite logically, entomologists and the faculty of agriculture schools consider that a large fraction of their research is directed toward application. Conversely, while it was to be anticipated that 48 percent of all life scientists employed outside the academic world engage in applied research, the fact that 79 percent of all such scientists consider that they are engaged in some fundamental research was somewhat surprising. It indicates that the prejudices of many young scientists against careers outside the academic setting, for lack of opportunity to engage in basic research, may well be ill founded.
Types of Research Conducted by Some Life Scientists.
In any case, the reader will recognize that there is no meaningful close definition of the terms “basic” and “applied” in these regards and that these indications by our respondents reflect their motivation in addressing specific problems and not the character of the work. By this measure, one investigator studying sodium transport in human erythrocytes may classify it as “basic” research; another may consider the same study “clinical,” only because human cells are employed for the purpose; and a third may view it as applied, since he hopes to develop a new drug. Taking into consideration these broad caveats, the data of Table 19 provide a useful description of the world of biological research.
- FINANCIAL SUPPORT OF RESEARCH IN THE LIFE SCIENCES
Research in the life sciences is a substantial national enterprise in which the United States invested $2,264 million in fiscal year 1967*; of this, 30 percent was provided by industry, 4.1 percent by foundations and other private granting agencies, 1.2 percent by academic institutions from their own resources, 0.3 percent by local and state governments, and 60.3 percent by the federal government, principal patron of the endeavor. Table 20 summarizes federal expenditures for life science research in fiscal year 1968. Research supported by industry was largely conducted in-house. In all, biomedical research conducted within federal laboratories required the expenditure of approximately $435 million. In part because of the proprietary nature of industrial biomedical research, and largely because the “principal investigator” in industrial and federal laboratories functions with a large supporting organization for whose expenditures he is not responsible, it was patently impossible to obtain, by questionnaire, meaningful data concerning research expenditures from individual scientists in these two sectors. Our data, therefore, are restricted to information provided by individual life scientists employed by academic institutions and by academic department chairmen. Only the former are considered in this chapter; the latter are discussed in the succeeding chapter. The collected data, summarized in Tables 21 , 22 , and 23 , indicate that in fiscal year 1967 the 4,046 responding academic life scientists, each of whom was principal investigator of one or more research grants or contracts, had available to them, collectively, $162,883,000 in support of the direct costs of research. The growth of this system is indicated by the fact that, in the previous year, the same investigators had available $134,726,000 and, in the prior year, $115,319,000. It is most unfortunate that we have no data for the same group in fiscal years 1969 or 1970, and, hence, no realistic data base with which to examine the consequences of the alterations in federal funding of science that have occurred since our questionnaires were distributed.
Federal Obligations for Research in Life Sciences, by Agency and Discipline—Fiscal Year 1968 (In Thousands of Dollars).
Financial Support of Academic Research in the Life Sciences (In Millions of Dollars).
Numbers of Research Grants and Contracts Awarded to 4,046 Academic Life Scientists.
Average Size of Research Grant (Direct Costs) in Thousands of Dollars.
It will be seen that, using our categorizations of the life sciences, molecular biology and biochemistry commanded one fourth of all reported support, a substantial fraction of which went to individuals with appointments in clinical departments. Following, in rank order, were physiology (17 percent) and disease mechanisms (14 percent). Only 1 percent of the total support went to scientists who stated that they were studying morphological problems and 2 percent, each, to those engaged in behavioral biology and in the study of systematic biology and evolution, with other research areas distributed in between.
The magnitude of support reported for the research area of disease mechanisms is disturbing in that, proportionally, it is very significantly under-represented. While the relative support per research area for all other areas may be considered a reasonably fair indication of the fraction of total national support that they command, this is surely not the case for disease mechanisms, presumably due to the disproportionately low response to our questionnaire by clinical investigators. Thus, it is highly doubtful that the support of research directly concerned with disease mechanisms by the National Institutes of Health is only 15 percent of its extramural research program, since half of its total extramural research support is granted to clinical investigators.
Caution is necessary in interpreting these data, however, because of the failure of the questionnaire to be sufficiently precise in guiding the respondents. Although “disease,” broadly taken, is the concern of clinicians and pathologists, there are no aspects of the study of disease, other than access to human patients, that are unique to their endeavors. In addressing himself to cardiac disease, the clinician may actually function as a physiologist who studies vector cardiography or analyzes the composition of blood obtained by catheterization of one of the cardiac chambers; or he may be concerned with the etiology and pathogenesis of atherosclerosis and so utilize the techniques and understanding of the biochemist or nutritionist. Concerned with a hereditary disorder, he may consider himself a human geneticist; if studying changes in the architectonics of the brain, he may view himself as a morphologist or even a student of evolution. If engaged in elucidation of the causative agent of an infectious disease, he may function, variously, as a cell biologist or a biochemist, while, if he is testing a drug in the hope of finding a successful therapeutic procedure, he is, at least for the time being, a pharmacologist. Accordingly, it is entirely possible that students of disease, its etiology, pathogenesis, incidence, or therapy, may well have indicated that their current research area lies in some category other than “disease mechanisms,” thus unintentionally distorting the interpretation that might be applied to these data.
The pattern of support from the National Science Foundation contrasts with that from the National Institutes of Health. Both supported molecular biology and biochemistry more heavily than any other category, but, whereas the National Institutes of Health also contributed in a large way to the study of physiology and disease mechanisms, the National Science. Foundation was clearly the principal supporter of systematic biology. The Atomic Energy Commission and the Department of the Interior, while contributing only 4 percent and 1 percent, respectively, to the total support of these life sciences, were particularly concerned with ecology. The principal thrust of support by the National Aeronautics and Space Administration, which contributed only 1 percent of the reported federal total, was in physiology, while only the Department of Agriculture and diverse industrial contributors allocated as much as one seventh of their research funds to studies involving nutrition.
Of interest is the fact that, whereas the voluntary societies were organized to combat the dread diseases, only 22 percent of their funds went to scientists who classified their own research as bearing directly on disease mechanisms, whereas one third of their support went to investigators in molecular biology and biochemistry, and one seventh each to studies of physiology and cellular biology. Clearly, the administrators of these societies were sufficiently understanding of the problems involved in treating and preventing these diseases to recognize the need for relevant basic research.
Table 21 indicates clearly that indeed the federal government is the principal patron of these areas of scientific endeavor. Three fourths of all funds in direct support of research derived from the federal government, while one sixth of such funds was provided out of the academic institutions' own resources. The low figures quoted for support by state and municipal agencies refer to direct granting activity, but the state budgets for the public universities contributed in major degree to the 16 percent of all directly research-supporting funds that are stated to have come from the institutions' own resources.
Particularly disappointing is the low order of contribution to research support provided by industry, private foundations, voluntary societies, and individual contributors shown in Table 21 . This is the consequence not so much of a low frequency of granting activity as it is of the relatively small awards actually made by these sources, as shown in Tables 22 and 23 . Thus, the average grant from industry was only $4,000, that from the voluntary societies, $10,000, and that from private foundations, $13,000. These figures are in contrast to grants from the National Science Foundation ($14,000), the National Institutes of Health ($30,000), and the federal average of $25,000.
Of some interest is the pattern of support by discipline. Typical grants in nutrition, ecology, and systematic biology are of the order of $15,000 per year, whereas grants to investigators in most of the other research areas were about twice as large.
Utilization of Research Grants
Typically, a research grant is utilized to provide consumable supplies, major and minor equipment, salaries of technicians and clerical staff, travel and publication costs, stipends for graduate students, postdoctoral fellows, and visiting investigators, as well as a variable fraction of the salary of the principal investigator not to exceed that fraction of his annual effort invested in the research project in question. Uniquely, research grants to clinical investigators may require expenditures in support of the basic costs of maintaining patients in hospitals; other grants may provide for unusual purposes such as ship time, international travel either to meetings or for work in the field, and, increasingly frequently, computer time. The relative distribution of expenditures among these various areas from research grants in support of research in the life sciences was not ascertained by the present study. However, data describing the general patterns of funding by the National Science Foundation are summarized in Table 24 .
Utilization of Funds from an Average Two-Year Research Grant in the Life Sciences—National Science Foundation—1968.
Research Support as a Function of the Investigator's Age
In a general way, increasing research support comes to the academic investigator as he gains seniority in the system. As shown in Figure 34 , this is clearly true for investigators supported by the National Institutes of Health and most other sources. The figures shown for “all sources” represent the simple arithmetic means for all grants from all sources. Because of the relatively large number of small grants from the National Science Foundation, industry, foundations, and voluntary societies, the mean grant size for all sources is decidedly less than that shown for the National Institutes of Health. Nevertheless, the trend is quite apparent: individual research support attains a maximum at 50 to 60 years of age and declines thereafter. This phenomenon is scarcely visible for the National Science Foundation, largely because this beleaguered agency strives to stretch its available resources as far as it can to support all qualified applicant investigators whose proposals fall within its purview, thus markedly reducing the amount of money available per applicant investigator.
Research support of life scientists as a function of their age. (Source: Survey of Individual Life Scientists, National Academy of Sciences Committee on Research in the Life Sciences.)
- RESEARCH INSTITUTES
The preceding survey of the major parameters of the world of biological research fails to convey the myriad arrangements for both research and education in biology. It ignores the dozens of small research institutes in which excellent investigators quietly pursue their research, occasionally with profound impact on the conceptual development of biology. The Cold Spring Harbor Laboratory for Quantitative Biology has had a brilliant record of achievement, and its summer courses have trained virtually all those who have led the modern development of virus and bacterial genetics, a major segment of molecular biology. Developmental biology and some aspects of neurophysiology have received great stimulus from the research and education programs of marine-biology stations such as that at Woods Hole, Massachusetts. Much of the current understanding of neurochemistry and the physiology of the brain has been obtained at small research institutes under private or state auspices, while ecology has grown at a multitude of field stations remote from their parent institutions.
- NATURAL HISTORY MUSEUMS
Natural history museums, with their combinations of scientists, research collections, and field stations are unique non-degree-granting academic institutions for research and graduate training. Quite apart from its role in public education through exhibits, a natural history museum contributes to the acquisition of scientific knowledge in two principal ways.
Its staff of scientists may engage in original research in systematic biology, evolutionary biology, ecology, geophysics, astrophysics, oceanography, and many other fields of science, depending upon their academic training and scientific interests. While many museum scientists depend on specialized collections in conducting their investigations, an increasing number engage in field and laboratory experimental studies of living organisms, or of ecological problems in natural settings. Their collections provide the basis for taxonomic-classification services necessary to many other scientists and also provide a base line for ecological studies.
The combination of resident scientists, research collections, and field research facilities provides intellectually attractive settings for visiting scientists. The number of graduate students who receive part or all of their graduate training in natural history museums is impressive and increasing.
Natural history museums, as both forums and research settings for systematists, ecologists, and environmental scientists, are becoming increasingly important as a national scientific resource, despite a long history of public neglect.
- BIOLOGICAL DISCIPLINES
For brevity and conciseness, we found it useful to structure all the life sciences into a dozen research areas. But this should not conceal the rich and diverse infrastructure of the life sciences. As we have seen, classical disciplinary labels have lost their meaning, but one could readily describe a hundred or more subdisciplines based on the work of groups of likeminded scientists who have blended the approaches of several older disciplines in attacks on some specific subsets of biological problems. A few examples are cited in the following paragraph.
Photobiologists, well versed in optics and the physics of light, are variously concerned with the mechanism of vision, the events in photosynthesis, the emission of light by bacterial and animal forms (the biological purpose of light emission by all but fireflies being not at all evident), and the photoinactivation of enzymes and viruses. Neuroscientists bring the skills of electrophysiology, cellular biology, molecular biology, and communications theory to bear on studies of information processing in the nervous system. Oncologists, focusing on the essential nature of the transformation of normal cells into malignant ones, are similarly a group apart, borrowing from every major discipline that may be of help, while vascular physiologists necessarily borrow from hydrodynamics and studies of urban traffic flow as they study the operation of a capillary bed or a major blood vessel. Physical anthropology is a subdiscipline that contributes to the total endeavor while it provides a bridge from the biological to the social sciences. It is the study of the bodily manifestations of human variation—in particular, the description of human body size, shape, and function in the light of man's history—and the role of heredity, environment, and culture in bringing about man's present diversity. The biological anthropologist aims to understand human physical variation and to apply his knowledge for human betterment through medicine and engineering.
As concern with the environment grows, an increasing number of physicians and biologists of many backgrounds have generated the area of research and practice called “environmental health,” the concern of one of the panels of this survey. More sophisticated understanding of this field should permit society to enjoy the fruits of an advancing technology, a superior living environment, and freedom to develop a society with fewer restraints and tensions. Past effort is minuscule compared with the magnitude of the problem. Since the problems increase with increasing population density and developing technology, efforts at controlling the environment, and thus the health of the population, must keep pace. Indeed, in a very real sense, students of environmental health serve technology by providing the knowledge permitting its benefits to be enjoyed without adventitious adverse effects on the health of man and, more broadly, on the environment of man. Thus, support of an adequate level of competence in environmental health is indispensable to a society that elects to make optimal use of the fruits of technology. Accordingly, the environmental-health resources of the nation must first be expanded to catch up with the problems now with us and thereafter be developed, along with technological development, to provide an adequate preventive program. Current support of research in environmental health probably lies between $30 million and $50 million per year; support for training for both research and practice is between $9 million and $18 million per year and is known to support (in 1969) 974 candidates for the master's degree, 981 candidates for the Ph.D., and 148 postdoctoral fellows.
A broad federal policy is needed, with a long-range plan of attack upon the whole problem of environmental deterioration and with better identification of the separate missions and responsibilities of the several federal departments and agencies. Only with such a policy will it be possible to develop in an orderly way the required training programs to supply the personnel needed for both research and practice, both within and outside the government, necessary to build a strong foundation for effective control programs against environmental-health hazards, a foundation that must rest on the entire current understanding of the life sciences.
Thus, the world of research in the life sciences is marvelously diverse. Tens of thousands of scientists in a thousand institutions contribute to its progress. They migrate between institutions, between classes of institutions, and between subfields of biology. They are quick to seize upon any new instruments or techniques, without regard to whether these are initially devised for use in the physical sciences or for some other research area in the life sciences. Biochemistry has become the language of biology, providing the bridge to the physical sciences, but it has yet to be applied to the farthest reaches of organismal biology. The federal government is the principal sponsor of the entire endeavor and, for the indefinite future, only the federal government can sponsor an effort of this magnitude. Its success will affect all aspects of our lives, and its conduct has become one of the central purposes of our civilization.
Basic Data Relating to the National Institutes of Health 1969, Associate Director for Program Planning and Evaluation and the Division of Research Grants, National Institutes of Health. U.S. Government Printing Office, Washington, D.C., 1969, p. 4.
The Invisible University: Postdoctoral Education in the United States, Report of a Study Conducted under the Auspices of the National Research Council, National Academy of Sciences, Washington, D.C., 1969.
Science Citation Index; An International Interdisciplinary Index to the Literature of Science. (Published by Institute for Scientific Information, Philadelphia.)
- Cite this Page National Academy of Sciences (US) Committee on Research in the Life Sciences. The Life Sciences: Recent Progress and Application to Human Affairs: The World of Biological Research Requirements for the Future. Washington (DC): National Academies Press (US); 1970. CHAPTER THREE, THE WORLD OF BIOLOGICAL RESEARCH.
In this Page
Recent activity.
- THE WORLD OF BIOLOGICAL RESEARCH - The Life Sciences THE WORLD OF BIOLOGICAL RESEARCH - The Life Sciences
Your browsing activity is empty.
Activity recording is turned off.
Turn recording back on
Connect with NLM
National Library of Medicine 8600 Rockville Pike Bethesda, MD 20894
Web Policies FOIA HHS Vulnerability Disclosure
Help Accessibility Careers
Thank you for visiting nature.com. You are using a browser version with limited support for CSS. To obtain the best experience, we recommend you use a more up to date browser (or turn off compatibility mode in Internet Explorer). In the meantime, to ensure continued support, we are displaying the site without styles and JavaScript.
- View all journals
Biological sciences articles from across Nature Portfolio
Biological sciences encompasses all the divisions of natural sciences examining various aspects of vital processes. The concept includes anatomy, physiology, cell biology, biochemistry and biophysics, and covers all organisms from microorganisms, animals to plants.
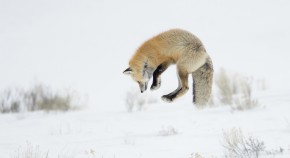
Streamlined skull helps foxes take a nosedive
Some fox species leap up and pounce head first into snow to capture prey that they hear below the surface. An analysis of the forces involved reveals how the shape of the skull has evolved to minimize damage from this behaviour.
- Mary Abraham
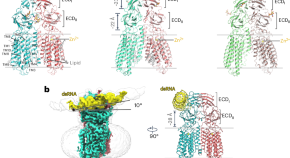
Cryo-EM structure reveals how SID-1 recognizes dsRNA that initiates systemic RNAi
Systemic RNA interference (RNAi) in Caenorhabditis elegans is initiated by SID-1-mediated double-stranded RNA (dsRNA) internalization. By combining cryo-electron microscopy (cryo-EM), in vitro and in vivo assays, we show how SID-1 specifically recognizes dsRNA and provide important insights into dsRNA internalization by SID-1.
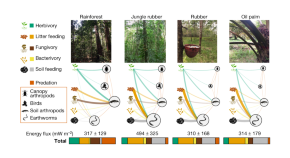
Changing rainforest to plantations shifts tropical food webs
A study provides insights into how energy flows in the food webs that connect soil- and canopy-dwelling organisms in tropical ecosystems with high biodiversity. When rainforest is converted to plantations, food webs are simplified and restructured, leading to profound changes in tropical-ecosystem functioning.
Related Subjects
- Biochemistry
- Biological techniques
- Biotechnology
- Cell biology
- Chemical biology
- Computational biology and bioinformatics
- Developmental biology
- Drug discovery
- Microbiology
- Molecular biology
- Neuroscience
- Plant sciences
- Structural biology
- Systems biology
Latest Research and Reviews
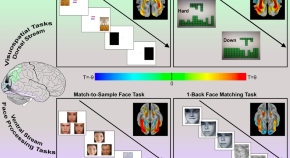
Contrasting neurofunctional correlates of face- and visuospatial-processing in children and adolescents with Williams syndrome: convergent results from four fMRI paradigms
- Madeline H. Garvey
- Tiffany Nash
- Karen F. Berman
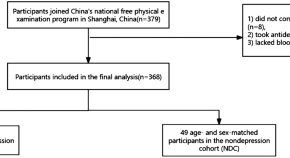
Study on plasma metabolomics profiling of depression in Chinese community-dwelling older adults based on untargeted LC/GC‒MS
- Jiangling Guo
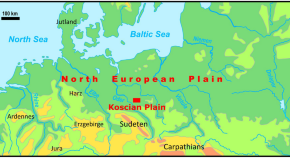
Permanent areas and changes in forests, grasslands, and wetlands in the North European Plain since the eighteenth century—a case study of the Kościan Plain in Poland
- Beata Medyńska-Gulij
- Krzysztof Szoszkiewicz
- Łukasz Wielebski
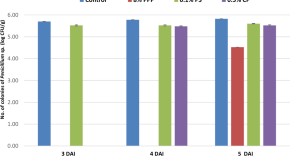
Comparative study on effect of pomegranate peel powder as natural preservative and chemical preservatives on quality and shelf life of muffins
- Namrata Ankush Giri
- Aditi Bhangale
- R. A. Marathe
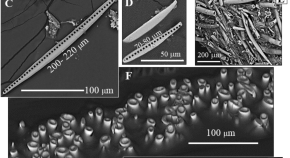
Biomineral crystallographic preferred orientation in Solenogastres molluscs (Aplacophora) is controlled by organic templating
- J. D. Castro-Claros
- W. W. Schmahl
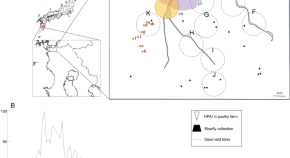
Blowflies are potential vector for avian influenza virus at enzootic area in Japan
- Ryosuke Fujita
- Takuji Tachi
- Shinji Kasai
News and Comment
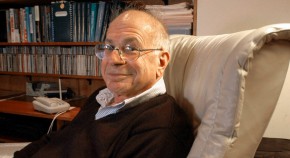
Daniel Kahneman obituary: psychologist who revolutionized the way we think about thinking
Nobel prizewinner whose insights into the foibles of human decision-making launched the field of behavioural economics and sent ripples through all social sciences.
- Eldar Shafir
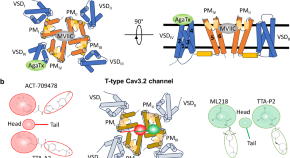
A tale of two calcium channels: structural pharmacology of Cav2.1 and Cav3.2
- Laurent Ferron
- Gerald W. Zamponi
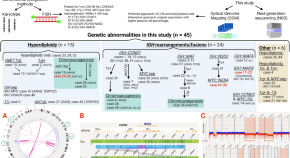
A comprehensive approach to evaluate genetic abnormalities in multiple myeloma using optical genome mapping
- Ying S. Zou
- Melanie Klausner
- Guilin Tang
Quick links
- Explore articles by subject
- Guide to authors
- Editorial policies

- Visit the University of Nebraska–Lincoln
- Apply to the University of Nebraska–Lincoln
- Give to the University of Nebraska–Lincoln
Search Form
Research areas.

Within the School of Biological Sciences, faculty are affiliated with one of two broad research areas. Faculty collaborate extensively within and between these areas as well as with colleagues in other departments.

Ecology, Evolution, & Behavior (EEB)
Faculty members address fundamental, interdisciplinary research questions that bridge ecological, evolutionary, physiological, and behavioral processes. We use a diversity of approaches to address these questions including: field studies, laboratory experiments, mathematical modelling, comparative genomics, functional genetics, systems biology, and phylogenetics. We are an interactive community of researchers who collaborate among laboratories and across the university to make new discoveries related to the diversity of the natural world.

Genetics, Cellular, & Molecular Biology (GCMB)
Faculty members use proteomic and genomic strategies, combined with bioinformatics to study fundamental biological processes of organisms ranging from viruses, prokaryotes, unicellular eukaryotes and fungi, to whole plants and animals. Areas of focus include cell biology, population and molecular genetics, epigenetics, microbiology, virology, immunology, structural biology, animal/plant pathology, genomics, bioinformatics, and molecular evolution. Interactive clusters of research groups focus on plant and animal virology, microbial physiology and cell biology, comparative pathobiology, plant growth and development, biotic stresses, biofuels, protein engineering, evolutionary genomics, and computational biology.
Faculty Research Areas of Interest
Animal Behavior Bioinformatics Biology Education & Science Communication Ecology Evolution Genetics & Genomics Human Health Mathematical Modeling Microbiology Molecular & Cellular Biology Parasitology Plant Biology & Pathology Virology
Animal Behavior
Bioinformatics, biology education & science communication, genetics & genomics, human health, mathematical modeling, microbiology, molecular & cellular biology, parasitology, plant biology & pathology, follow and engage with us.

Meetings help us connect with our peers and have insightful discussions. ASCB Photo.
Often in our scientific careers we are faced with the question of how to choose an area of research to pursue. As a graduate student picking a laboratory in which to do a PhD, a postdoctoral researcher wanting to pursue a career in science, or even as a principal investigator running a laboratory, we are sometimes required to focus or change direction to a new area of biological research. These decisions may be daunting as we have to devote a significant number of years in our chosen research field and it is important to be confident about the field before diving in with a lot of time and money.
Below are a few tips to help with picking a research area!
- Read scientific literature: Published scientific literature gives a good idea about the research field and the big unanswered questions that are left to be studied. Scientific reviews on the topic are often useful to understand the big discoveries in the field and the anticipated future studies that will provide more information. It is also important to know if the research area has sufficient unanswered questions that will be interesting to funding agencies. Try to understand if the field has long-term potential. Scientists often work on certain research areas for decades and so thinking ahead about hypothetical questions and probable answers is one key to success.
- Attend conferences/seminars: Attending both large and small meetings help us connect with our peers and have insightful discussions. Meetings also have poster sessions on various topics that may be useful to learn about the different research areas out there. Such meetings are also a good place to learn about technical details or new experimental strategies, which are often important when forging into a different field.
- Brainstorm ideas with peers: When looking for a research laboratory it is important to find something that interests you. Working on an interesting question will help you go the extra mile and aid in making significant discoveries. Talk to your peers about their experiences and the pros/cons in their research field. Peers can also help review research grants and their experience and perspectives may provide useful feedback.
- Define focused questions in the research area: Research areas can be very broad. It is easy to digress into multiple directions without focus. Before diving into the research, decide on a few hypotheses and preliminary experiments. Having more than one hypothesis will be important in case the primary hypothesis does not hold. Once experiments work and the project progresses, remember to stay focused. As part of your scientific growth, learn to think of tangential experiments that may be useful projects for other members of the laboratory.
- Ensure the research is fundable: To continue doing research it is essential to have funding. Before delving into the research define the significance of the proposed research. It is always useful if discoveries can, in the future, cure or treat diseases. Significance also helps us explain our research to nonscientists and family, so that they can relate to the research and understand what we study. Public outreach will help get more funding and aid in conducting more research.
Ultimately research has to pique your interest and stimulate answers to tough questions. Good luck choosing a research laboratory or changing scientific directions!
Comment below if you have additional suggestions!
About the Author:
Recommended articles.

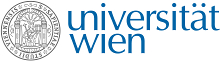
- Show search form Hide search form
- Quick links
- Staff search
- Search Search --> Websites Staff search Start search
Key Research Areas
Research at the faculty of life sciences focus on the fundamental understanding of biological systems, their organisation and evolution. curiosity-driven basic research in biology, pharmacy and nutritional sciences provides the basis for application-oriented research to find sustainable solutions to global challenges and problems of the society. the faculty of life sciences defines 8 key research areas., anthropogenic evolution.
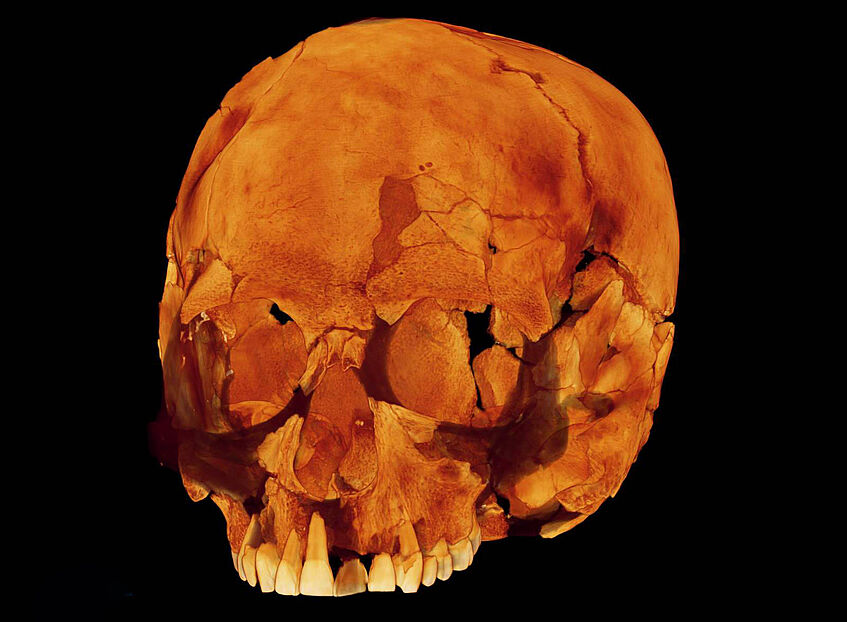
Biomolecules for a Healthy Lifespan
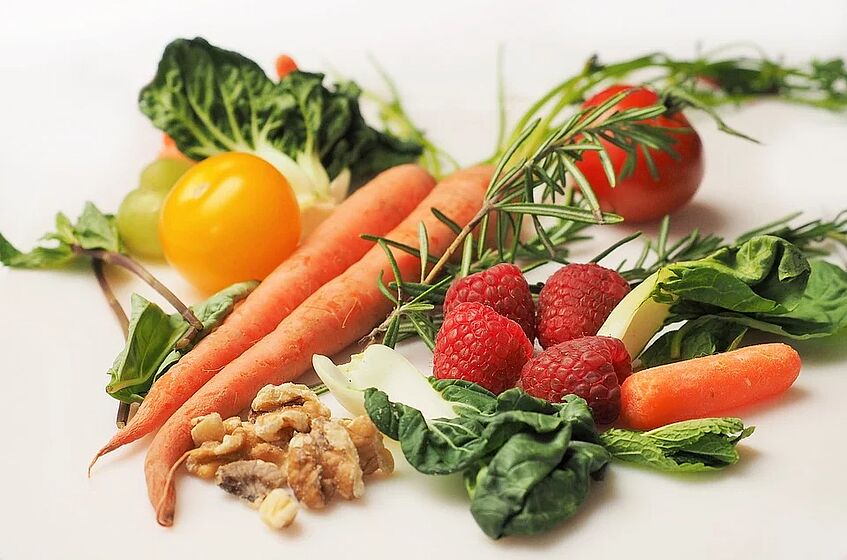
Cognition, Behavior and Neuroscience
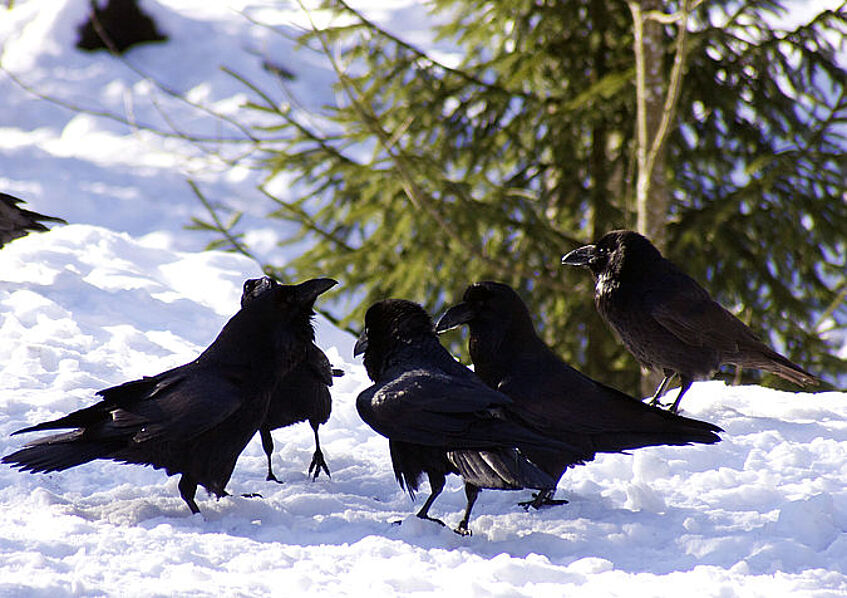
Computational Life Sciences
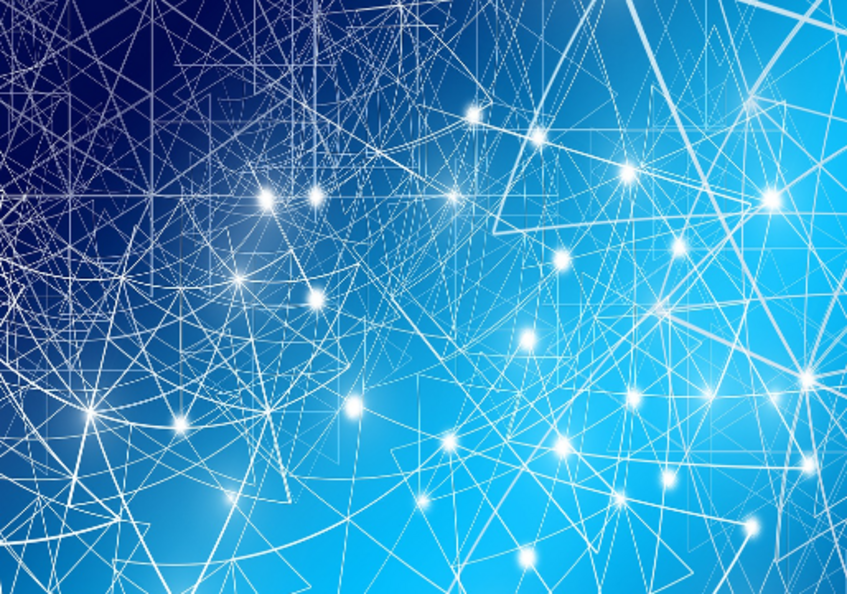
Environmental Change Ecology, Biodiversity and Sustainability
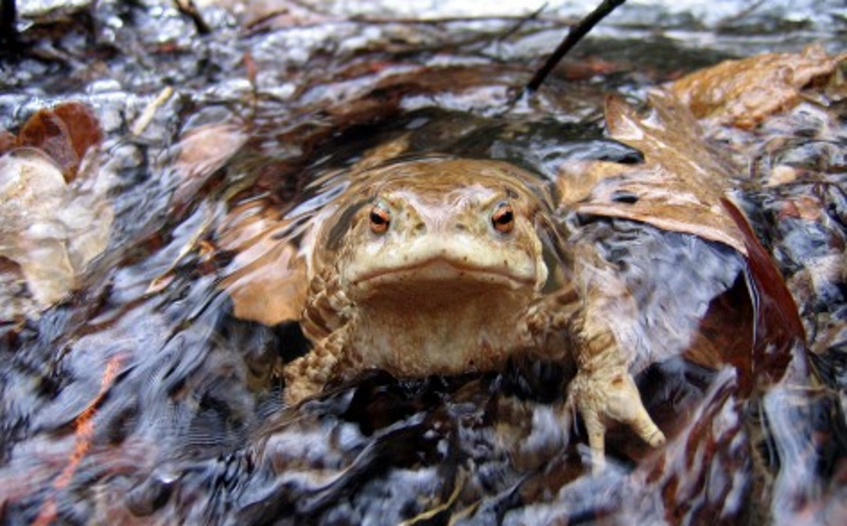
Green Planet – from Genes to Ecosystems
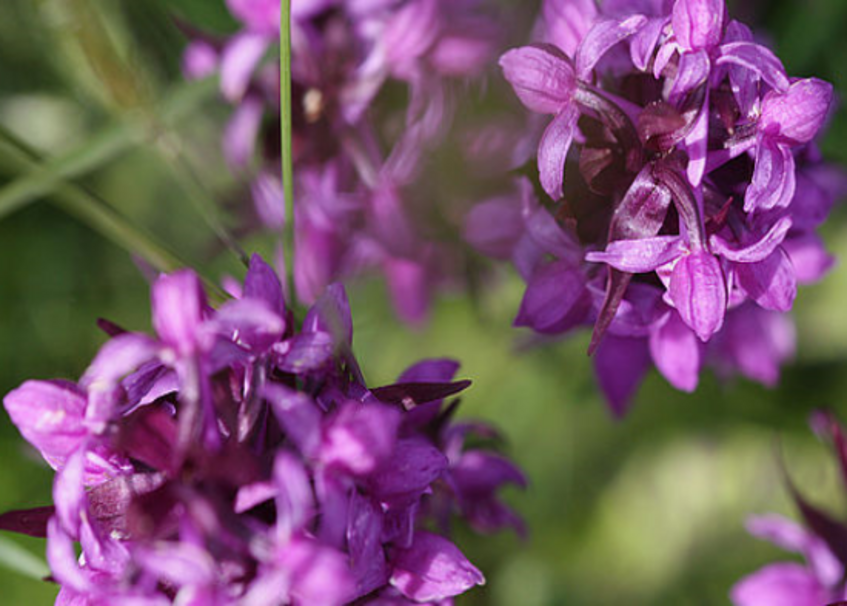
Innovation in Drug Research
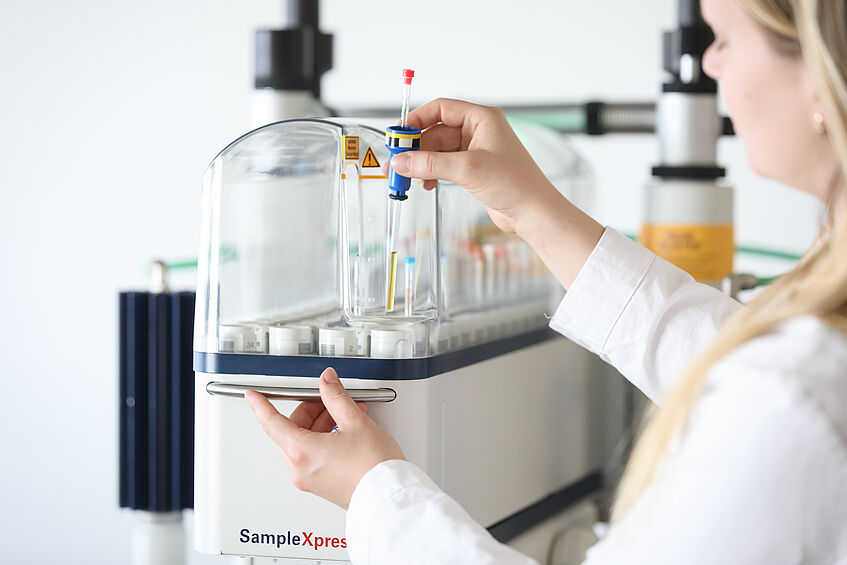
Interactions and Evolution of Organisms
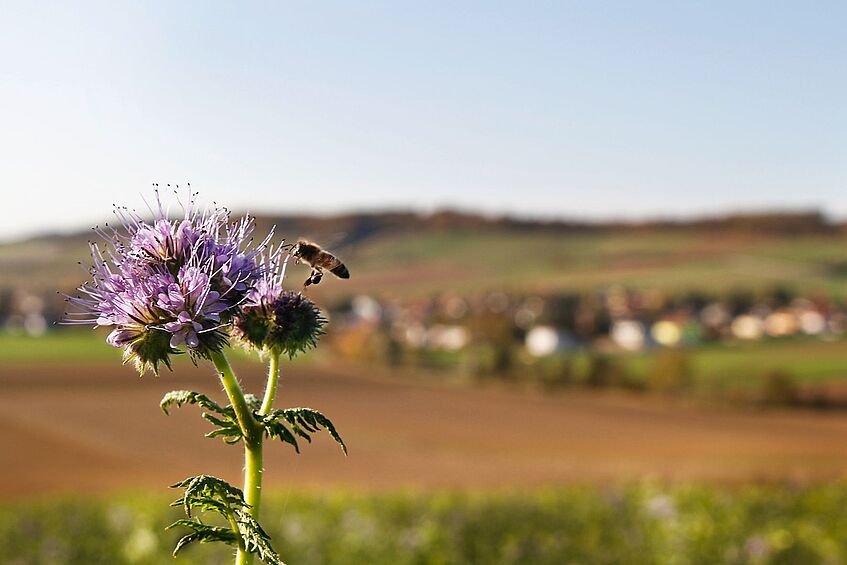

- Faculty of Biological Sciences
- Research degrees
- Studying with us
Areas of research
The Faculty is home to more than 100 academic researchers supervising over 250 PhD and MSc by Research students, studying subjects across the full spectrum of biology from ecosystems to molecular machines. We have an extensive list of research areas;
- Biotechnology
- Cardiovascular
- Cell and Organismal Biology
- Ecology and Evolution
- Heredity, Development and Disease
- Integrative Membrane Biology
- Microbiology
- Neuroscience
- Plant Sciences
- Sport and Exercise Sciences
- Structural Biology
Listen to some of our current research students and leading academics talking about some of our key research areas and what projects they are working on that match their passions and will enable them to make a real difference;
Biomedical research
Ecology and Evolution research
Plant Science research
Heredity, Development and Disease research
Department of Biological Sciences
- Home ›
- Faculty & Research ›
Areas of Research
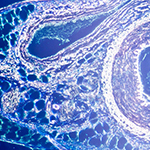
Anatomy & Physiology
Now that sequencing whole genomes is possible, the next big problem is to understand what those genes do. This is the realm of anatomy (study of the structure of tissues, organs, and organ systems) and the closely related field of physiology (study of how those structures work). Researchers in the department study adaptations to subzero temperatures, circadian control of metabolism, neuroendocrinology, evolution and pathobiology of the mammalian skull, and reproductive biology. Many of our physiologists bridge the molecular and the organismal.
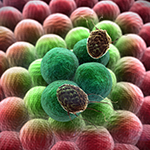
The cancer biology program draws upon a variety of distinct and complementary approaches to better understand the molecular, cellular and genetic determinants that govern tumor formation and disease progression. Utilizing a combination of experimental in vitro and in vivo models as well as clinical samples, our researchers investigate fundamental mechanisms that govern tumor initiation, cancer cell migration and invasion, tumor cell survival, metastasis, cell cycle control, the tumor microenvironment and chemoresistance. Many projects bridge basic research with clinical platforms for cancer diagnostics and treatment.
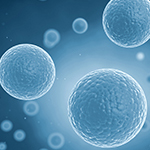
Cellular & Molecular Biology
Cell biology is the study of cells, the basic structural unit of all living systems. Our researchers seek to understand how cells work as individual units with a focus on membrane and cytoskeleton organization, how they organize into complex groups, how they coordinate their activities in organismal systems and how they respond to extracellular signals and infectious agents. Dissecting how cells function at the molecular level is becoming increasingly important for understanding the cellular basis of human disease.
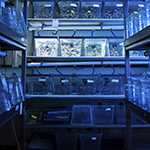
Development & Regeneration
Investigators in our department use a variety of model systems to answer fundamental questions in developmental biology and regeneration. Our researchers examine the mechanisms that direct cell growth, differentiation, organogenesis and regeneration of tissues such as blood, heart, retina, and kidney, relevant to our understanding of human development and disease. Researchers are also using innovative molecular approaches to understand the biology of stem cells, such as to uncover the genetic pathways that regulate induced pluripotent stem cells and factors that govern the behavior of tissue-specific stem cells in the body.
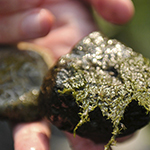
Ecology & Environmental Biology
Profound changes are affecting our environment, from climate change to habitat loss, pollution, and invasive species. Understanding ecosystems and their function is critical to crafting a sustainable future for humans and the planet. Our researchers study the responses of organisms to the environment and the interactions among species in ecological communities. We work in terrestrial and aquatic ecosystems in all corners of the world, from the savannas of Africa to the grasslands and rivers of Alaska and the Arctic. Laboratory and mathematical experiments augment field studies, and many researchers apply their research in management and policy.
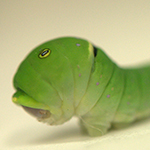
Most of the world’s animals are insects. Insects play a critical role in ecosystem function, many of them transmit dangerous disease, and some of them are endangered by environmental change. Researchers from across all areas of the department work with insects, sometimes as models for basic physiology and development, sometimes to understand infectious disease, and sometimes to study population dynamics and the interaction of species such as herbivores and host plants. As a result, the university has excellent facilities for rearing insects, and core facilities for the study insect genomes.
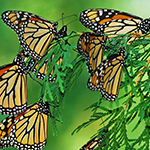
Epidemiology & Population Biology
Modeling of the many layers of information in biological systems has long been a mainstay of biology investigation. As the magnitude and quality of data grow exponentially, epidemiological modeling of the key features of disease have become pivotal to the discovery process in our efforts to understand and combat infectious diseases. Furthermore, the mathematical descriptions of populations have been empowered by modern genomic approaches that give us fine-resolution views of genome organization and its impact on disease genetics, geographic variation and the spread of both pathogens and alleles that are the focus of several Notre Dame faculty.
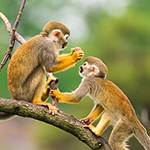
Evolutionary Biology
Organisms are evolving and changing every day, creating, molding, and even deleting genetic diversity. Meanwhile, next-generation sequencing is reinventing evolutionary biology and our ability to track and probe evolutionary processes. Our researchers use cutting-edge tools to understand evolutionary processes within whole genomes that lead to differences in organismal function. We also use evolutionary differences to detect species in nature and predict their responses to environmental change. We study the evolution in many organisms, mostly in wild populations, including human diseases and their hosts.
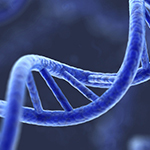
Genetics & Genomics
Notre Dame Biology faculty are engaged in a wide range of genetic studies that leverage the inheritance patterns (chromosomes and genes) that confer important traits. Modern genomic tools and approaches, including whole genome sequencing and 'transcriptome' analysis, are dramatically empowering the search for the genes and mutations that determine diseases, development, drug resistances and even natural variation and speciation. Notre Dame has established both the instrumentation and interdisciplinary analytical facilities to support these expansive and revolutionary views of biological systems at the resolution of molecular mechanisms.
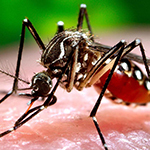
Infectious & Vector-Borne Diseases
Researchers investigate the cellular and genetic determinants of infectious diseases with emphases on the host response to infection, modeling and epidemiology of disease and mechanisms of transmission and prevention. The program is highly collaborative, interdisciplinary and bridges basic sciences with comprehensive educational and research endeavors to help build integrated programs in global health.
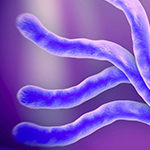
Microbiology & Immunology
Microbiology is the study of single-celled and multi-celled microscopic organisms. Many microorganisms cause dangerous infectious diseases. Immunology is the study of the immune system in a diversity of organisms. Microbiology and Immunology go hand in hand, as microbes that cause disease trigger an immune response and manipulate the immune system during infection. Researchers work with bacterial and eukaryotic microorganisms and viruses, which cause a range of diseases including Dengue Fever, Malaria, Leishmaniasis, and Tuberculosis. They are also interested in understanding how the immune response in host organisms responds to infection.
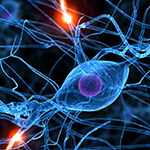
Neuroscience & Behavior
Neuroscience is an interdisciplinary field that considers problems from multiple perspectives, including molecular level processes and complex social behaviors. Our researchers work in the areas of sensory neurobiology (especially vision and olfaction), stress and pain processes, circadian rhythms, control of social behaviors, and computational neuroscience. We collaborate with colleagues in the departments of chemistry and biochemistry, psychology and anthropology, among others.
Apply to the Graduate Program
Loading metrics
Open Access
Essays articulate a specific perspective on a topic of broad interest to scientists.
See all article types »
Key attributes of successful research institutes
Roles Conceptualization, Investigation, Supervision, Writing – original draft, Writing – review & editing
* E-mail: [email protected] (FB); [email protected] (SAT)
Affiliation Laboratory for Axon Growth and Regeneration, German Center for Neurodegenerative Diseases (DZNE), Bonn, Germany

Roles Writing – review & editing
Affiliation Wellcome Sanger Institute, Hinxton, Cambridge, United Kingdom
Affiliations Wellcome Sanger Institute, Hinxton, Cambridge, United Kingdom, Cavendish Laboratory, University of Cambridge, Cambridge, United Kingdom
- Frank Bradke,
- Aidan Maartens,
- Sarah A. Teichmann
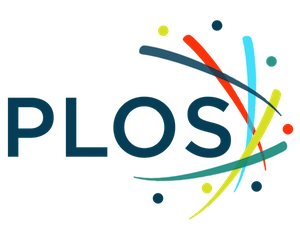
Published: September 5, 2023
- https://doi.org/10.1371/journal.pbio.3002267
- Reader Comments
Science does not take place in a vacuum: The physical and social workplace has a profound influence on scientific discoveries. Everyone at a research institute can contribute to its scientific output and productivity, from faculty research groups to facilities and platforms staff to administration and corporate services. Although the researchers addressing exciting scientific questions are key, their efforts can be fostered and directed by the overarching strategy of the institute, interconnection with facilities and platforms, and strong and directed support of the administration and corporate services. Everybody counts and everybody should be empowered to contribute. But what are the characteristics that make scientific organizations and their people flourish? This Essay looks at the structure and culture of successful research institutes, laying out different operational strategies and highlighting points that need be taken into consideration during their implementation.
Citation: Bradke F, Maartens A, Teichmann SA (2023) Key attributes of successful research institutes. PLoS Biol 21(9): e3002267. https://doi.org/10.1371/journal.pbio.3002267
Copyright: © 2023 Bradke et al. This is an open access article distributed under the terms of the Creative Commons Attribution License , which permits unrestricted use, distribution, and reproduction in any medium, provided the original author and source are credited.
Funding: The authors received no specific funding for this work.
Competing interests: The authors have declared that no competing interests exist.
Introduction
When scientists start working at a research institute, they quickly realize that they are not just inside the bubble of their own laboratory, but are part of a bigger ecosystem. In the words of John Donne, “no man is an island,” and this rings true of scientists and research groups as well. The culture of a research institute, its scientific standards, its social cohesion, and its funding framework are critical to its research output. But what are the key ingredients for a thriving institute?
Research institutes across the globe have put considerable efforts into building environments that facilitate the conception and exploitation of novel scientific ideas. A critical aspect of these environments is educational: throughout their careers, scientists continuously learn from each other by emulation, discussion, collaboration, and competition. This reflects the proverb that states “it takes a village to raise a child.” A research institute provides exactly this. It is the whole village—with all its constituent residents—in which scientists develop, formulate, and pursue their ideas, but also from which they emerge to join other scientific communities worldwide.
In this Essay, we explore strategies that have helped institutes develop into particularly successful and special places. This analysis is based on our almost 4 decades of experience working as group leaders and program heads at research institutes and in sitting on institutional scientific advisory boards and external review panels. These experiences spurred hours of discussion among ourselves and with colleagues, mentors, and mentees about the underlying philosophy, research culture, and organizational structures of successful, adaptable, and forward-looking research institutes. Here, we share our perspectives from these informal discussions. There is an inevitable geographical bias towards those institutions that we have interacted with in one way or another, but we hope the lessons we have gleaned have relevance to research institutes across the globe.
Research institute organization and culture
The philosophy behind most research institutes is to free up scientists’ time to focus on research, with little or no teaching and the provision of internal funding. Many institutes do run world-class postgraduate training programs in affiliation with universities, but the focus tends to be on training through research projects rather than theoretical lectures. Research institutes often encourage interdisciplinarity and collaboration, and many form a structure to promote the intersection of disciplines such as biology with technologies and methods rooted in physics, chemistry, or computer science, for example. Research institutes have been remarkably successful, as demonstrated by their major contributions to ground-breaking discoveries such as the classification of developmental patterning genes [ 1 ], the determination of high-resolution biomolecular structures using cryo-EM [ 2 , 3 ], and the discovery of the structure of the ribosome [ 4 , 5 ], to name just 3 examples.
Research institutes occupy a specific niche in the larger research ecosystem. Their success can be measured by scientific contributions in the form of novel ideas, publication output, and grant funding. Furthermore, success is assessed not by shareholders but primarily by other scientists, both in a broad community sense and in terms of advisory boards, funding bodies, and so on. Other measures of success include the satisfaction levels of staff and trainees, their career development to go on to contribute to society in research or other venues, and commercial impact, for example. Selected examples of research institutes, their histories and characteristics can be found in Box 1 .
Box 1. Research institutes and their characteristics
The success of the research institute model is exemplified by biomedical research institutes. One prominent example is the Laboratory of Molecular Biology (LMB) in Cambridge, United Kingdom, which was established by the Medical Research Council in 1947 and was the PhD training ground and dozen-year long workplace for one of us (SAT). The LMB boasts 12 Nobel Prizes and revolutionary breakthroughs such as key contributions to the discovery of the DNA double helix structure. Similarly famous is the European Molecular Biology Laboratory (EMBL), whose successful PhD program was the training ground for another one of us (FB) and boasts 3 Nobel Prizes. This intergovernmental research organization was founded 1974 and provides amazing opportunities to group leaders to perform ground-breaking research through generous core funding in a completely free, blue skies research environment. As an example of a more recently established institute, the Howard Hughes Medical Institute (HHMI) Janelia Research Campus in Virginia, United States of America was opened in 2006 and has an interdisciplinary approach ranging from mechanistic cognitive neuroscience through to the new “4D cell physiology” program. Its philosophy is to create a culture of collaboration with freedom to pursue research.
Many institutes have a dominant scientific and/or technological culture, be it structural biology (the LMB), genomics (the Wellcome Sanger Institute in Hinxton, UK), or neuroscience (HHMI Janelia Research Campus or the German Center for Neurodegenerative Diseases (DZNE) in Bonn, Germany). In addition, a “founding myth” and research culture, often related to the original leadership, is often woven into the institutes’ identity. For example, John Sulston propagated an open science and open data spirit at the Wellcome Sanger Institute, with a strong emphasis on team science, whereas Paul Nurse has built the Francis Crick Institute in London, UK into an environment supporting both basic and translational research and fostering the collaboration across both areas. The LMB identity is often associated with decades-long dedication to a single large scientific challenge, exemplified by Max Perutz’s solution of the structure of hemoglobin; additionally, Perutz’s manner of interacting with LMB colleagues in an informal, open, and nonhierarchical way feeds through to the institute’s culture today.
An important question that has grown in importance in recent years is how research institutes can promote a positive research culture. To us, research culture includes how staff and trainees interact with each other across the institute, what the institute defines as valuable and important, and what its definitions of success are (e.g., discoveries, papers, grants, spin-offs, training the next generation). It is influenced in both top-down and bottom-up ways: Leadership can set the tone and the values of the institute, while younger researchers, if well-integrated and listened to, can influence the institute’s direction and give the institute energy and excitement. It is also not just about people: Positive research culture can be incubated by the physical structure of the institute, in venues for social eating, sports, and after-work socializing, for example, as well as by regular events such as retreats to promote team building and coherence. The bottom line is that research culture is not just an added benefit for an institute: it can either nurture or impede the creativity of individual scientists, and as this creativity drives scientific discoveries, institutions are increasingly taking it seriously (see [ 6 , 7 ] for insightful discussion).
Thus a key question is how to incubate an inspirational research culture where productivity, ambition, and high-quality science are encouraged in a balanced, supportive, and inclusive way. While scientific output is generally perceived as the result of work by bench research scientists alone, there are multiple structures within a research institute involved in research delivery, and thus everyone in an institute is part of the research mission and should be recognized for their contributions. What are the ingredients required to craft a successful, collaborative, supportive, and thriving research environment? Below, we outline some key interacting components, which are summarized in Table 1 .
- PPT PowerPoint slide
- PNG larger image
- TIFF original image
https://doi.org/10.1371/journal.pbio.3002267.t001
Considerations for a successful research institute
While there is not a single recipe for an ideal institute, every aspect of the “village” can influence the progression of the next generation of scientists and the discoveries they produce. Core facilities, a supportive administration and research groups form the golden triangle of a well-run institute ( Fig 1 ). Within the golden triangle, research institutes need to consider the following concepts to maximize their success (explored in detail in the following sections):
- Listen, inside and out. Institutes need feedback mechanisms, both internal and external, to continuously evolve and optimize their organization and science.
- Enable scientists to focus on the science. Institutes benefit from effective, proactive, and communicative underpinning administration with a deep understanding of research culture, to free up scientists to focus on their science.
- Promote “plug and play” research. Effective and agile facilities and operational infrastructure, with state-of-the-art of the equipment, can be tremendously enabling for research.
- Build a holistic research environment. The institute needs a supportive research culture that empowers scientists to develop and realize their potential and promotes creativity.
While institutes are run in different ways and have different purposes, consideration of these key ingredients will help them to thrive.
Institutes are built on a framework of funding and governance indicated in the floor of the building, which can be varied depending on the institutional model. To build and maintain the institute, strategic recruitment is important, as are supportive and constructive advisory committees (shown on the left-hand side). For a research culture that fosters productive and creative science, continuous open communication and collaboration is key between the 3 basic components within the institute: faculty research groups, facilities and platforms, and administration and corporate services. Varied types of outputs of a successful research environment are shown on the right-hand side. (Illustration credit: Christina Usher.).
https://doi.org/10.1371/journal.pbio.3002267.g001
Listen, inside and outside: Effective feedback mechanisms
Effective feedback mechanisms help research institutes assess how well their progress is aligning with their goals. Perhaps the most important source of feedback to the leadership is from staff working within the institute, who will have direct knowledge about how well the institute is running on a day-to-day level. Open channels of communication for staff are thus vital: this could be to their direct line managers or as regular (and optionally anonymous) surveys. These can then feedback into internal assessments on a more formal level; for example, annual reviews can showcase not only scientific achievements but also policies regarding staff wellbeing that have been enacted following feedback.
One of the key external sources of feedback comes from funding reviews and assessments, which can focus more on the achievements of individual research groups (such as at the LMB or at the Max-Planck Society institutes in Germany) or on entire departments or programs (such as at the Wellcome Sanger Institute or at the Helmholtz Association institutes in Germany). The kind of assessment regime chosen may influence the science that happens in the institute. More individual-based reviews have the benefit that responsibility lies very clearly with an individual principal investigator (PI), but intra-institute collaborations are not specifically incentivized. Of course, individual groups can independently reach out to potential collaborators, but the institute leadership needs to closely monitor how such interactions and scientific collaborations develop. The leadership might also influence collaboration by implementing a PhD and postdoc program for training in the latest techniques in the different research areas or provide a venue for cross-pollination of different ideas to stimulate intellectual creativity and innovation. Crossing disciplines does come with risks as well as opportunities [ 8 ], especially if the groups do not speak a common language, and again leadership can step in in these situations, providing opportunities as well as monitoring the effectiveness of interactions (occasional joint review meetings with members of leaderships of across disciplines may help to facilitate this).
By contrast, the more collective-based review of entire departments or programs may incentivize intra-institute collaboration. This facilitates and expedites delivery of large research programs with a single common goal, as epitomized by the concerted contribution from the Sanger Institute to the Human Genome Project [ 9 ] and the Human Cell Atlas [ 10 ], and the Rhineland-Study at the DZNE [ 11 ]. To make sure that individuals from different fields will be motivated to interact with each other around the shared project in such collective programs, leadership needs to provide opportunities for interactions and ways of monitoring their effectiveness. Large projects with relatively well-articulated goals can catalyze development of technologies and provide data sets that individual laboratories can build on in the future (advances in genome sequencing during the Human Genome Project is a clear example of this).
Regardless of whether a research institute adopts individual or group evaluation, it is crucial to have a transparent and clear process that is communicated upfront to all parties within the institute and to the evaluation committee. The process should be proportional and efficient, as limited in time as possible, and occur with as little possible disruption to the research at the institute. In addition, a separate, carefully appointed standing scientific advisory board that engages in constructive feedback can provide vital and complementary comments. Such advisory boards ideally contain 1 or 2 members with long-term knowledge of the institute’s history and culture, who truly know the organization. Their sustained input can be vital, especially for new institutes still finding their feet. Finally, it is worth considering widening the net in terms of guidance and feedback to include wider society (for an example, see Box 2 ). We see engagement with wider society as a two-way dialog, with both parties benefitting [ 12 ].
Box 2. Examples of successful practices by research institutes
Expand feedback.
- The Centre for Genomic Regulation in Barcelona, Spain engages citizens and stakeholders to guide and co-create its long-term research strategy .
Support core facilities
- EMBL’s ARISE program of fellowships supports training of highly educated research infrastructure scientists. Researchers who want to stay in science but not on the tenure track are provided with an opportunity to learn the skills necessary to develop and maintain infrastructure, and core facilities will benefit from hiring well-trained staff.
- Cross-Europe initiative Core for Life brings together core facilities managers to together define best practices, provide training, validate and share technologies, and advocate for the importance of funding for core facilities.
Boost technology transfer
- Yeda commercializes the intellectual property generated by scientists at the Weizmann Institute in Rehovot, Israel.
- The Vlaams Instituut voor Biotechnologie (VIB) Innovation & Business team (Ghent, Belgium) bridges the institute’s science and industry by establishing patents, intellectual property licenses, industry collaborations, and spin-off companies.
- The Tri-Institutional Therapeutics Discovery Institute in New York, USA helps researchers from 3 biomedical centers translate their discoveries to preclinical studies.
Expand trainee experience
- Many organizations, such as EMBL , provide PhD training programs across departments/sites, increasing exposure to multiple disciplines.
Improve equity, diversity, and inclusion
- The Instituto Gulbenkian de Ciência in Lisbon, Portugal runs the António Coutinho Science Awards , which provide fellowships and research funding to citizens of Portuguese Speaking African Countries (PALOP) or descendants of those from PALOP countries.
- The Wellcome Sanger Institute recently launched the three-year postdoctoral Excellence Fellowships , which are aimed at people from Black heritage backgrounds.
- The Athena Swan charter can help institutions achieve gender equality objectives and provide structures for self-assessment.
- The Wellcome Sanger Institute has a Behavioral Competency Framework (BCF) that all employees sign up to and which defines the behaviors expected and encouraged in the entire institute.
- International graduate programs such as Taiwanese International Graduate Program foster diversity by recruiting PhD students from across the world.
Network across institutes
- EU-LIFE is an alliance of leading life sciences research centers from 15 European countries, which forms a strong advocacy voice to influence policy, share best practices, and inspire open and ethical science.
Let scientists focus on the science with a proactive and communicative administration
While all scientists love discussing their new discoveries and next experiments, and some also like to spend time developing longer term scientific strategies, very few are passionate about administrative organizational strategy. This may be a mistake given the importance of an efficient administration for the smooth running and success of a research institute [ 13 ]. While institutes rightly seek excellence in faculty recruitment, they should also invest effort into recruiting and training excellent administrators.
The administrative apparatus needs to balance the desire of scientific leaders for unconstrained freedom with the necessity of managing budgets and applying business principles and governance in often publicly funded, not-for-profit organizations with liabilities and legal obligations. Finding the balance between these 2 objectives is challenging. For administration to operate harmoniously with research groups, dedication to the research culture of the institute and a proactive approach to enable science are foundational. Administrative staff need to understand that speed and flexibility can make all the difference in science. Every employee joining the administration should be made aware of the scientific research process in the institute. What are the results, discoveries, and outputs of scientific research? Why does timeliness matter? Administrative staff should understand the importance of rapid and nimble responses when providing scientists with working instruments, tailored contracts, and efficient and understandable guidelines. Supportive administrative staff will proactively provide information and co-develop (with researchers) streamlined processes and standard operating procedures to help the scientists achieve their research goals while complying with rules and regulations.
A bottom-up, proactive administration culture can be usefully complemented by a top-down organizational strategy. The administrative leadership needs to clearly articulate the vision and overarching goal of rapid and nimble research support, empowering proactive and creative problem solving by all administrative staff members. A key element of this is the level of tolerance with respects to mistakes. Getting the balance right between agility, speed, and risk aversion is important for administrative teams.
To help achieve this mindset and culture, a “liaison” system between administrative team heads and faculty members can be remarkably effective. Each administrative department can have research group leaders who act as contact points, resulting in a bidirectional dialog and exchange of information. Similarly, when faculty members understand the mechanisms and constraints of administration, they can recognize when and how to request changes effectively—communication is vital.
To develop a proactive administration who are aligned with the research aims and culture of the institute, administrative staff must feel welcomed and valued. However, administrators commonly feel their skills are underappreciated [ 14 ]. Thus, it is key to communicate with respect and to publicly acknowledge and support the vital work that administrators do.
It is impossible to overemphasize the importance of communication to an institute. Internal communication has a key role in its day-to-day functioning: top-down, it allows all staff to stay informed of the long-term goals and strategies of the institute; bottom-up, it allows leadership to stay abreast of the research advances and practical concerns of the workforce; and sideways, across the institute, researchers greatly benefit from knowing what their neighbors are doing (this can help foster collaborations). Smooth and positive communication across roles enables a committed and effective research culture to flourish.
External communications, on the other hand, ensure that discoveries are not confined to the pages of scientific journals. As many institutes are publicly funded, they have a duty to convey their discoveries to the public in relatable and exciting ways. Thus, a well-supported communications office can be a great help to any institute. Communication professionals can act as the bridge between scientists and the media, help write press releases and create audiovisual output, and train scientists in how to tell their research stories. External communication also has a key role in defining an institute’s success and promise to potential funders. In certain regions, philanthropic donations can make up a significant portion of an institute’s income, and therefore, many institutes have dedicated philanthropy departments to manage and promote this activity. Here, a key aspect is donor engagement: the institute needs to communicate the impact of donations, which may be in the form of events, lay reports, or lab tours, for example.
Facilitate “plug and play” research with facilities and operational infrastructure
Core facilities, comprising technical instrumentation, methods, and the expertise of highly trained staff, enable faculty teams to focus on new areas of scientific development while benefitting from services such as next-generation sequencing, microscopy, bioinformatics, and research software engineering. Core facility teams often implement cutting-edge methodologies for the benefit of the whole institute [ 15 ], and shared core facilities can offer efficiencies and economies of scale. Indeed, core facilities can act as “force multipliers” for various essential aspects of the institute, from recruitment and retention to grants and knowledge production [ 16 ].
To develop the broad expertise needed to optimally exploit new technologies, community and collaboration are key. For example, faculty teams and core facility staff can work closely together to develop, optimize, and scale-up a new technique or methodology. Just as we discussed with regards to administration, it is crucial to get core facility staff on board with the vision of the institution and to publicly acknowledge their work, such as via authorship and/or acknowledgements in papers and talks, and in events or awards. Two examples of endeavors to support core facility staff are outlined in Box 2 .
The governance of core facilities is an important consideration: researchers must be ensured fair access, and a balance must be struck between adopting cutting-edge techniques and maintaining a high level of robustness and reliability in results and data delivery. A steering committee structure can maintain oversight of the facility, and a transparent online system can monitor services provided and usage statistics, turnaround times, and key performance indicators. Depending on the research focus of the institute, some facilities can grow to be exceptionally large and need a more defined structure and management. For example, research institutes that deal with large genomic data packages and huge sample sizes require specific adopted management schemes (for an informative case study, see [ 17 ]).
Build a holistic environment to empower individuals to develop and realize their potential
Research institutes are not merely spaces where science is done. Rather, they can proactively accelerate research advances and enhance the day-to-day lives and career prospects of the researchers within. This can be in more structural/formal respects (such as recruitment, technology transfer, and training) and also in social/cultural dimensions that generate a positive environment where ideas and creativity thrive.
Recruitment
Research is fundamentally about the people involved, so recruiting talented staff that contribute to the institute aims and culture is vital. Hiring decisions have far-reaching implications for the institute in terms of both science and culture, and this applies across the board for anyone hired in any capacity. Institutes deal differently with recruitment, ranging from an internally driven process (e.g., Wellcome Sanger Institute) to a largely external assessment (e.g., EMBL, where hiring committee members are drawn from across the international European Molecular Biology Organization membership). Each have their benefits and risks.
In internally driven recruitment, the institute and its leadership are in complete control of individual promotions, recruitment, science, and personnel strategy. The vision and strategy of the leadership team is executed in a top-down manner, with the advantage that decisions can be made quickly. At the same time, unchallenged views on strategy and personnel selection may allow bias and nepotism to creep into the process. To counterbalance this, human resources departments have a key role in ensuring well-managed recruitment campaigns, and the external scientific review process provides further checks and balances. External assessment has the advantage of objectivity and potentially avoiding personal issues and local politics. Recruitment by hiring committees with eminent external members may help increase trust in a system. Potential disadvantages include the risk of “parachuting” someone in who does not understand the research areas and organizational context of the current faculty and also a risk of consensus decisions that promote orthodoxy in hiring. There may not be a single ideal way of conducting recruitment in a given research institute—what is key is an awareness of the pitfalls of both approaches and taking steps to counterbalance them.
Once hired, scientists rightly want to be fairly renumerated for their efforts. While an attractive package is a nonnegotiable factor in decisions about where to work, extra perks such as on-campus childcare or residential accommodation can make a huge difference to the lives of scientists. Differences in pay within the institute need to be well justified based on experience and delivery. Maintaining fairness and transparency is crucial in order to avoid pay and resource gaps that are not justified based on scientific productivity, such as the gender pay gap (for a recent survey of this gap in the UK, see [ 18 ]). Finally, the option of dual hires (where spouses are hired to the same institution) can be a huge incentive for a candidate to accept an offer, particularly if they are coming from a different country.
A key question is whether to hire new PIs on tenure-track or no-tenure models. The dynamically changing workforce that no-tenure models can generate might avoid intellectual stagnation and help the leadership adapt to new challenges with new hiring. At the same time, new PIs may be put off by the lack of long-term security and be more attracted to tenure-track models. To counteract this, research institutes can extend time periods of fixed-term contracts and offer more generous core funding. In our experience, a good distribution of experience contributes to a stimulating research environment: junior group leaders bring energy, dynamism, and a fresh, sometimes revolutionary perspective, while senior group leaders contribute mentorship, experience, stability, and strategic perspectives. A healthy balance of faculty members with different levels of experience, without a concentration of only junior or only senior group leaders, may thus be a common aim.
The number of faculty is also a key consideration. There is no “ideal” institute size because more or less staff may be required depending on the research questions and funding environment. While smaller institutes may appear to provide a more convivial atmosphere, the social “tone” of an institute of any size can be influenced by leadership style and work culture, as discussed below. Related to institute size is group size. While some institutes restrict group size (e.g., the Francis Crick Institute and the LMB), others have no explicit limit. Small groups can have effective communication and teamwork, and their leanness can give a greater focus on a particular problem, whereas large groups are able to be interdisciplinary, with critical mass in 2 or more areas, and provide the benefits of research done at scale. Indeed, research as a whole flourishes with a diverse range of group sizes, with large groups expanding and building up research, and small groups starting new areas of inquiry [ 19 ].
Whatever the size, institutes work best when grouped around either a technology (e.g., the Structural Studies Division at the LMB) or around a biological topic (e.g., neurodegeneration at the DZNE). This leads to the question of how to manage the extent of interdisciplinarity within an institute. Focus on a single discipline might inhibit creativity, while too much interdisciplinarity might lead to a lack of overlap between research groups, with no benefit from synergies. The best space might therefore be somewhere in between [ 6 ].
Individuals are what make any institute run—science is really about people and their day-to-day interactions. This means that recruitment can make a huge difference to the “feel” of an institute. When hiring new faculty members, scientific capability is of course key, but not the only aspect. How well will this personality merge with the existing faculty? Alternatively, are they too similar, so would the institute benefit from disruption of the status quo? Do they fully buy in to the research culture and vision that the institute is striving for? Individuals with energy and drive can further energize others, provide inspiration for students and postdocs and, in turn, make the institute an attractive place to work for future hires. Identifying such individuals can thus be key, particularly in the early stages of an institute’s life.
The appointment of a new director is one of the most influential recruitment decisions, as they act as a figurehead for the institute, externally and internally. The new appointee might take the institute in an entirely new research direction or aim to develop a new kind of research culture: the appointment itself is a statement of the future vision of the institute. What counts as an ideal candidate will of course depend on an institute’s priorities. Is scientific excellence paramount or administrative experience? As discussed above, the decision between hiring from inside or outside will also have great implications. While an insider may understand the institute’s unwritten rules and be able to quickly identify key issues, an outsider may bring a fresher view, with novel ideas and management qualities. Personal style is crucial here, but overall leadership (of any organization) is fundamentally about serving the mission and the people in the institute. Directors who are exceptional in this regard have often been founders or early directors of institutes (e.g., Janet Thornton, an early director of the EMBL–European Bioinformatics Institute), perhaps because they feel so closely intertwined with the institutes’ identity and people.

Technology transfer and training
Once staff are in place, there are various ways that research institutes can build a supportive and inspirational research environment. As hubs of innovation, institutes generate novel ideas and inventions, and to ensure that these findings are protected and taken forwards for the benefit of society, a technology transfer system can manage intellectual property and the process of licensing and commercialization. Innovation and business development teams can also educate researchers to promote an entrepreneurial attitude, and institutes in turn must make space for, recognize, and reward activities such as the founding of start-up companies. This could be via reward and incentives through sharing of royalties from patents or the use of employment contracts with a percentage of time given over to entrepreneurial activity. Examples of successful technology transfer are shown in Box 2 .
In addition to helping scientists achieve impactful discoveries, forward-looking institutes will train scientists at all levels in the key skills required for the scientific workplace, but also in communication, research management, and leadership. Scientific training will allow researchers to stay up to date with cutting-edge methodologies. To prepare students and postdocs for life as an independent investigator (if this is the path they choose), research institutes can provide access to training in writing papers and grants, project and lab management, and science communication/public engagement. PhD programs that provide training across departments and sites can be extremely useful and stimulating, exposing students to various techniques and scientific perspectives early on in their training that are often exploited in subsequent thesis work and later careers. An institute’s success may be measured in large part by the impact of its trainees on society, and a well-supported training and outreach program can be invaluable to this.
The mentorship of younger scientists by more senior staff in the institute can be crucial to their career success. As well as providing career guidance, compassionate mentors outside of the host lab can help to tackle research misconduct in its many forms. Young researchers should have trust in the wider system and know that they can raise concerns without having to challenge their supervisor directly: a supportive mentoring network can provide this.
Building an inclusive environment
One of the beauties of working in science is encountering people from different cultural backgrounds, and research institutes should aim to integrate different personalities and characteristics, fostering diversity, and viewing it as a strength. An inclusive environment brings out the best in scientists and science [ 20 , 21 ], and different ways of thinking contribute to greater creativity. Thus, diversity in scientific approaches is more likely to be found among a group of diverse individuals, and a successful research culture takes equality, diversity, and inclusion seriously. As we have argued with respect to gender equality [ 22 ], this must be built in at the policy level so that the system is organized to incentivize good behavior. We are also encouraged by the efforts of funders in this regard, such as Wellcome [ 23 ] and HHMI [ 24 ]. Going forwards, research institutes need to further develop and promote inclusive policies encompassing areas such as equality of opportunity, parental leave policies, protection from bullying and harassment, and sustainability. There should be public, high-level buy-in for these policies and dedicated funding streams.
There have been numerous recent examples of research institutes implementing policies to promote equality, diversity, and inclusion in locally relevant ways ( Box 2 ). Ensuring diversity on management and advisory boards is another aspect that needs to be developed to achieve a greater diversity in the workforce at all levels in the long term.
The majority of discussions about diversity of science (inevitably including those covered in this piece) are centered around helping institutions in Western nations increase diversity. The risk is that this is less relevant for other institutes, particularly those in nations where English is not commonly spoken. For such institutes, a bigger problem may be to attract top science talent from the global marketplace. A highly funded institute in a wealthy nation may appear “more diverse” because it has the luxury of attracting global talent, and the definition of what constitutes “diverse” will differ from country to country. While we do not explore these issues further here, we hope this article spurs responses from colleagues around the world, who might share their local experiences for research institute success. Discussions with colleagues based in Taiwan show how institutes there have established international PhD programs ( Box 2 ) and targeted recruitment of faculty and guest professorships to access the global talent pool.
Wherever the institute, a positive research culture can be helped by a clearly articulated code of conduct ( Box 2 ). Such frameworks should be developed with the staff of the institute, should be open to change over time, and be flexible enough to accommodate the various cultural backgrounds and personalities that necessarily make up a modern research institute. Rather than being a restrictive straight jacket or homogenizing tool, they should be a way of recognizing and fostering behaviors that contribute to a positive research culture without suppressing personal authenticity and freedom of speech. Indeed, there are many different types of people that can be accommodated and contribute to a team. Exciting research often occurs on the fringes: There is a risk that research institutes stifle those with unorthodox views who may not appear to conform to standard expectations of behavior or research direction, yet bring unique strengths and perspectives to the table. The challenge for leadership is how to accommodate researchers with potentially difficult characteristics within a broadly inclusive and tolerant environment.
Finally, a positive research culture must have staff wellbeing at the core: just as an institute must ensure staff safety via a dedicated health and safety program, staff mental health should also be high on the radar of institute leadership. There are various ways in which mental health can be supported (for a recent perspective, see [ 25 ]).
Meeting today’s and tomorrow’s challenges
Research institutes today have to meet a host of new, often interlinked, challenges that stand in the way of their ability to transform society with scientific innovation. A first challenge is simply to get the necessary funding to carry out research. Many institutes have found their funding curtailed in recent years (e.g., due to austerity programs that reduce government spending on research or inflationary pressures). To help counter this, prominent institutes can act as vocal advocates for science funding. A well-run communications department and publicly vocal and compelling director will undoubtedly help in this regard. Institutes can and should be in the public conversation, helping to counter disinformation and increase public trust in science, which ideally will feed back to politicians allocating budgets. Elsewhere in the world, research funding has been a persistent issue that will likely continue into the future. While philanthropy or global funding sources are potential solutions, another option is to expand international collaborations between institutes (taking inspiration from EU-LIFE, see Box 2 ). In this way, people, skills, and knowledge would move between countries in a way that strengthens local capacities. Is the time ripe for a United Nations of Research Institutes?
The COVID-19 pandemic was a global challenge that changed the way all research institutes worked. While the short-term effects were seismic—institutes shut for months at a time during lockdowns, freezing whole programs of research—there were also more lasting impacts, notably the naturalization of hybrid or remote working. This brings its own challenge: how can we foster team cohesion when interactions are mainly virtual? Online conferences are often less interactive than in-person ones, leading to fewer of the chance meetings that seed many of the innovations of the future (for discussion, see [ 26 ]). The risk is that the same might occur within research institutes, if the corridors and cafeterias are not filled with the sounds of scientists discussing their latest data. On the other hand, the virtualization of science can increase inclusivity, both at local and global levels (e.g., those with caring duties can work from home, while cutting-edge science can be shared virtually worldwide to expand knowledge transfer). Overall, for an institute, maintaining a strong identity and research culture may always rely on people meeting in person, but harnessing new virtual technologies to increase remote interactions (e.g., in virtual reality) can also complement this. A great challenge in the future therefore is how to maintain the physical–virtual balance.
A global challenge that will only increase in coming years is the climate crisis. Many scientists are increasingly uncomfortable with flying for work (for discussion, see [ 27 ]). Institutes can also have a role here, for example, in mandating the number of international trips expected from faculty as part of wider carbon reduction or net zero policies. A slew of climate-related societal challenges will inevitably impact research institutes, including food shortages, changes in migration patterns, acute health impacts, and physical impacts on infrastructure such as flooding. The institute’s operational leadership will need to keep these impacts on the horizon to guide their choices. Just as important will be the psychological effects of a changing world on the people working within the institute: climate anxiety may, at least in part, be mitigated by strong and open climate policies at the institute level.
A final challenge—and opportunity—is the increasing inclusion of artificial intelligence (AI) into an institute’s work and culture. While it is still difficult to envision how AI will affect our approaches, we expect that we are at the beginning of a major change. For example, the newly developed ways to analyze data and literature using AI may provide transformative insights for research and research culture. We expect AI to change how we develop novel scientific ideas and concepts. Institute leadership needs to closely follow the challenges and opportunities that arise through this transformative technology, and importantly, involve all staff in conversations about how best to move forward.
Overall, a research institute has to balance a multitude of factors. It needs to support scientists to flourish and forge a career, while simultaneously adopting an overarching research strategy that relies on good team workers, efficient project/research managers, and good leaders of people. Holistic, collaborative, and responsive approaches are vital to create scientific villages that foster the next generation of scientists and continue to produce world-changing discoveries.
Acknowledgments
We thank Genevieve Almouzni (Institut Curie), Mary Barlow (EMBL-EBI), Monica Bettencourt-Dias (Instituto Gulbenkian de Ciência), Ansgar Büschges (University of Cologne), Hwai-Jong Cheng (IMB, Academia Sinica), Martin Dougherty (Wellcome Sanger Institute), Eugenio Fava (DZNE), Yukiko Goda (RIKEN), Ilona Grunwald Kadow (University of Bonn), Muzlifah Haniffa (Wellcome Sanger Institute), Sabine Helling-Moegen (DZNE), Reinhard Jahn (Max Planck Institute for Multidisciplinary Sciences), Sarah Jewell (DZNE), Yishi Jin (UCSD), Lizzy Langley (Wellcome Sanger Institute), Cheng-Chang Lien (National Yang Ming Chiao Tung University), Pierluigi Nicotera (DZNE), Steve Palmer (Wellcome Sanger Institute), Maggie Payne (Wellcome Sanger Institute), Mike Stratton (Wellcome Sanger Institute), Gaia Tavosanis (University of Aachen), Ron Vale (UCSF), and Dagmar Wachten (University of Bonn) for reading and discussion. We thank Christina Usher for the figure illustration.
- View Article
- PubMed/NCBI
- Google Scholar
- 9. Wellcome Sanger Institute Press Office. The first draft of the Book of Humankind has been read. Wellcome Sanger Institute [Internet]. 2000 Jun 26 [cited 2023 Jun 20]. Available from: https://www.sanger.ac.uk/news_item/first-draft-book-humankind-has-been-read/ .
- 10. Wellcome Sanger Institute Media Team. International Human Cell Atlas Initiative. Wellcome Sanger Institute [Internet]. 2016 Oct 14 [cited 2023 Jun 20]. Available from: https://www.sanger.ac.uk/news_item/international-human-cell-atlas-initiative/ .
- 11. JPND Research. The Rhineland Study. JPND Global Cohort Portal [Internet]. 2017 Sep 21 [cited 2023 Jun 20]. Available from: https://www.neurodegenerationresearch.eu/cohort/the-rhineland-study/ .
- 24. Howard Hughes Medical Institute. HHMI’s Commitment to Diversity, Equity, and Inclusion. HHMI [Internet]. 2022 [cited 2023 Jun 20]. Available from: https://diversity.hhmi.org/?_ga=2.10400117.1732995758.1642680506-849369497.1630417893 .
Cancer Biology Research
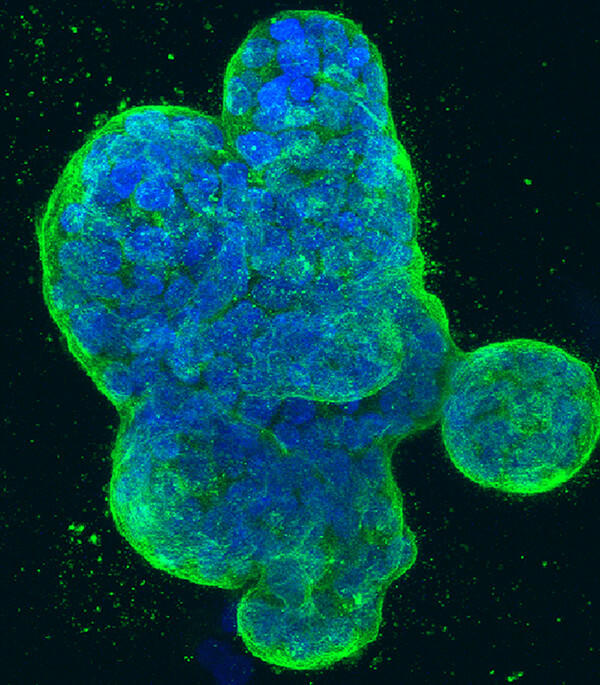
Breast cancer cells
The Importance of Cancer Biology Research
Research on the biology of cancer starts with the simplest of questions: What is—and isn’t—normal? To understand how cancer develops and progresses, researchers first need to investigate the biological differences between normal cells and cancer cells. This work focuses on the mechanisms that underlie fundamental processes such as cell growth, the transformation of normal cells to cancer cells, and the spread ( metastasis ) of cancer cells.
Virtually all major advances against cancer originated with discoveries in basic science . Basic research can reveal new ideas about the causes of cancer and how it develops, progresses, and responds to therapy.
Knowledge gained from such studies deepens our understanding of cancer and produces insights that could lead to new clinical interventions. For example, studies of cell signaling pathways in normal cells and cancer cells have contributed greatly to our knowledge about the disease, revealing molecular alterations that are shared among different types of cancer and pointing to possible treatment strategies.
Decades of basic research in cancer biology have created a broad base of knowledge that has been critical to progress against the disease.
Selected NCI Activities in Cancer Biology Research
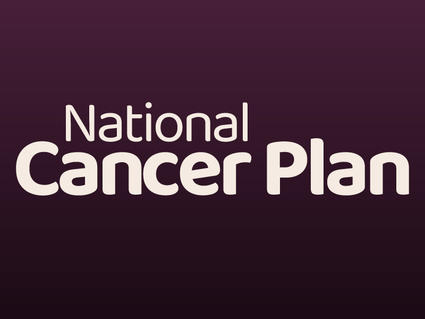
NCI Research and the National Cancer Plan
NCI supports a broad variety of research that aligns with the goals of the National Cancer Plan. Read about the plan and explore each goal.
Federal funding for cancer biology is essential because this area of research receives relatively little funding from entities that are driven by profit. NCI supports and directs cancer biology research through a variety of programs and approaches. For example:
- The Metastasis Research Network (MetNet) supports research to improve our understanding of how cancer spreads. Cancer metastasis is a complex, dynamic, nonlinear process. The network supports several specialized centers working collaboratively on multidisciplinary projects focused on several themes of the metastatic process, including mechanisms of early dissemination, cellular and physical microenvironment crosstalk, dormancy, and mechanisms of responses to therapy by metastatic cells.
- The Translational and Basic Science Research in Early Lesions (TBEL) Program is advancing the understanding of the mechanisms driving, or restraining, the development of precancers and early cancers, as well as informing the development of precision prevention approaches. The program supports multidisciplinary research centers that are integrating basic and translational research to investigate the interactions of an early lesion, its microenvironment, and host factors as “co-organizers” of tumor initiation and the development of cancer.
- The Human Tumor Atlas Network is constructing 3-dimensional atlases of the cellular, morphological, and molecular features of human cancers as they evolve from precancerous lesions to advanced disease. The atlases, which represent a diverse patient population, will also be used to study how tumors respond to treatment and develop resistance to drugs.
- The Cancer Tissue Engineering Collaborative (TEC) supports the development and characterization of state-of-the-art biomimetic tissue-engineered technologies for cancer research. This program advances innovative, well-characterized in vitro and ex vivo systems available for cancer research, expands the breadth of these systems to several cancer types, and promotes investigations of cancer with tissue-engineered systems.
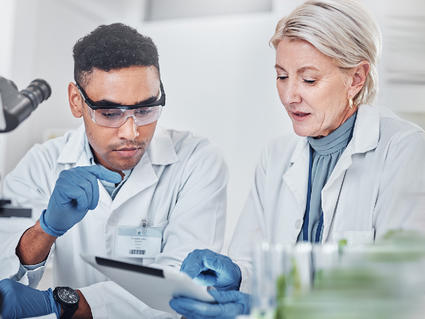
NCI Fiscal Year 2025 Professional Judgment Budget Proposal
Each year, NCI prepares a professional judgment budget to lead progress against cancer.
- The consortium of tumor glycomics laboratories and their research partners that make up the Alliance of Glycobiologists for Cancer Research are investigating the cancer-related dynamics of complex carbohydrates. The alliance, which NCI sponsors with the National Institute of General Medical Sciences and the National Heart, Lung, and Blood Institute, aims to study the structure and function of glycans in relation to cancer.
- The NCI RNA Biology Initiative facilitates the exchange of information and expertise among investigator studying the structure, function, and biological roles of RNA for the purpose of developing new cancer diagnostics and therapies.
- NCI’s Centers of Excellence bring together the institute’s intramural researchers to collaborate on new projects and initiatives in various areas of cancer biology, including Chromosome Biology and Genitourinary Malignancies .
Recent Research Findings in Cancer Biology
- Loss of Y Chromosome in Men Makes Bladder Cancer More Aggressive
- Cells’ decision to divide is reversible
- How Fatty Liver Disease Helps Cancer Thrive in the Liver
- No Glucose? Pancreatic Cancer May Have a Ready Energy Alternative
- How Some Brain Tumors Hijack the Mind to Grow
- Researchers discover the multiple shapes of RNA, a boon for drug design
- Vulnerability in Brain Tumors May Open Door to New Treatments
- Preventing Chemo Brain? Study Identifies Potential Approach for Common Problem
Cancer Biology
Focus areas.
The mission of the Department of Cancer Biology is to identify and understand the causes of cancer, to develop innovative approaches to reduce cancer incidence, to create and test novel and more effective therapies, and to translate these findings into clinical care for the benefit of patients.
Research in our department is highly collaborative and is potentiated through close interactions with other basic science departments and translated through clinical collaborations.
Our faculty members are aligned into eight research focus areas that address cancer development, progression and treatment.
Cancer disparities
While cancer affects everyone, certain groups have higher rates of cancer cases, deaths and health complications. These differences can be associated with genetics, sex, racial and ethnic populations, socioeconomic status, or specific geographic areas. Studying the factors that lead to cancer disparities leads to more-effective prevention and treatment approaches for the affected populations.
- Read more about the cancer disparities focus area .
Cancer stem cells
The stem cell theory of cancer proposes that among the many different types of cells within a cancer, there exists a subpopulation of cells called cancer stem cells that multiply indefinitely, are resistant to chemotherapy, and are thought to be responsible for relapse after therapy. Cancer stem cells also give rise to highly metastatic cells that spread to other organs and tissues within the body. A deeper understanding of cancer stem cells is leading to better implementation of existing anti-cancer therapies and identification of new approaches that target the cancer stem cells specifically.
- Read more about the cancer stem cells focus area .
Cancer systems biology
Cancer is a complex disease with many molecular, genetic and cellular causes. Considering these causes as a system of interactions can lead to a better understanding of the processes involved in the development of cancer and response to therapies. Cancer systems biology integrates advanced experimental models, insights from genome sequencing and other large-scale data projects, and computational models to create unified models of cancer behavior.
- Read more about the cancer systems biology focus area .
Oncogenic gene dysregulation and carcinogenesis
Cancer initiates from genetic alterations, including mutations, deletions and copy number gains, that function to activate cancer-promoting pathways or to block processes that normally inhibit cancer development. Through better understanding of pro- and anti-cancer signaling processes and how they become dysregulated in carcinogenesis and tumor progression, our team can improve biological tools for clinicians and devise molecularly targeted therapies to intervene.
- Read more about the oncogenic gene dysregulation and carcinogenesis focus area .
Precision cancer medicine and translational therapeutics
Cancers develop and respond to therapies differently from one patient to the next. A better understanding of the specific processes driving cancer growth and spread in an individual patient allows for tailored therapeutic strategies that are more effective with minimal side effects. Profiling the mutations and abnormalities that drive a tumor, in combination with development of experimental models that assess the specific responses of cancer cells to therapeutics that target those abnormalities, has dramatically improved outcomes in many cancer types.
- Read more about the precision cancer medicine and translational therapeutics focus area .
Tumor immunology and immunotherapy
Therapeutic strategies that stimulate the immune system to target cancer cells can lead to long-lasting tumor regression and minimize relapse. Integrated efforts of laboratory researchers and clinicians are leading to improved knowledge of how the immune system interacts with cancer cells and how immune processes can be intentionally manipulated for therapeutic effect.
- Read more about the tumor immunology and immunotherapy focus area .
Tumor invasion and metastasis
A fundamental property of malignant tumor cells is the ability to invade surrounding tissues and to metastasize to other organs. These abilities underlie the majority of cancer-associated deaths. While invasion and metastasis are often thought of as the final stages of tumor development, recent studies have shown that tumor spread can occur even at early stages of tumor development, sometimes even before the primary tumor has been identified. Development of therapies targeting invasion and metastasis has the promise to significantly reduce cancer mortality.
- Read more about the tumor invasion and metastasis focus area .
Tumor microenvironment
Tumors require complex interactions with surrounding blood vessels, immune cells, supportive tissue structures and cell types that are distinct to the tumor site in order to grow, become invasive and metastasize. Tumors influence their microenvironment by releasing soluble signals that lead to degradation and remodeling of the tissue structures that constrain their growth. Targeting the interactions of tumors with the microenvironment is an important and developing area of study.
- Read more about the tumor microenvironment focus area .
More about research at Mayo Clinic
- Research Faculty
- Laboratories
- Core Facilities
- Centers & Programs
- Departments & Divisions
- Clinical Trials
- Institutional Review Board
- Postdoctoral Fellowships
- Training Grant Programs
- Publications
Mayo Clinic Footer
- Request Appointment
- About Mayo Clinic
- About This Site
Legal Conditions and Terms
- Terms and Conditions
- Privacy Policy
- Notice of Privacy Practices
- Notice of Nondiscrimination
- Manage Cookies
Advertising
Mayo Clinic is a nonprofit organization and proceeds from Web advertising help support our mission. Mayo Clinic does not endorse any of the third party products and services advertised.
- Advertising and sponsorship policy
- Advertising and sponsorship opportunities
Reprint Permissions
A single copy of these materials may be reprinted for noncommercial personal use only. "Mayo," "Mayo Clinic," "MayoClinic.org," "Mayo Clinic Healthy Living," and the triple-shield Mayo Clinic logo are trademarks of Mayo Foundation for Medical Education and Research.
Marine Biology
- College of the Environment
- University of Washington
Core Research Areas
There are many ways to focus your studies or research in marine biology, and it can be difficult to know where to start. The University of Washington has faculty with expertise in a diverse range of research areas related to marine biology. To help you explore these areas, we group many topics of interest into four ‘core research areas’. Click on a specific topic to find faculty who teach or research in the areas you’re interested in.
Many Marine Biologists research specific groups of organisms. How and why did they evolve? What is unique about this group of organisms? Many people have an early interest in sharks, whales and fish. Try exploring a group you aren’t as familiar with – lesser-known groups such as marine invertebrates or seaweeds may surprise you!

Marine Microbiology Salmon Sharks Fish Invertebrates Marine Mammals Seabirds
How do organisms in the marine environment move, get energy, or reproduce? How do they adapt to the stresses of their environment? How do they interact with each other? When we examine processes, we think about the physiology of an organism (i.e, how does it work?) as well as how that organism is similar to or different from other life in the ocean. This can advance our understanding of life in the sea, and may even have implications for human health or engineering.

Evolution & Adaptation Animal Behavior Genetics/Genomics
Habitats and Ecosystems
Marine life does not exist alone. It is part of a complex system of interactions with other organisms and the physical environment. Studying the ‘big picture’ through ecology or oceanography is a critical part of marine biology.

Salish Sea Ecology Tropical Ecology & Corals Oceanography Arctic Ecology Quantitative Ecology & Modeling
Changing Oceans
The oceans are constantly changing due to the natural cycles of tides or seasons to longer-term changes in global climate. Humans influence change in the oceans as societal and economic forces drive what we take out of the ocean and what we put in. Marine Biologists have an important voice in decisions about conservation and marine policy.

Science Communication Resource Management Climate & Global Change Conservation

- Research at Vienna BioCenter
- Research Entities
Anthropogenic Evolution
Biochemistry, biophysics, structure, cell and chromosome biology, cognitive and behavioral biology, computational biology, genomes and evolution, disease mechanisms, immunology, pathogens, gene regulation, rna, epigenetics, microbiology and environmental systems science, neurobiology, plant sciences, stem cells, development, regeneration.
- Research Groups
- CRISPR/Cas9
- MECHANISMS OF CHROMOSOME SEGREGATION
- HUMAN BRAIN ORGANOIDS
- EPIGENETICS
- 1001 GENOMES
- Recent Research Highlights
- Austrian BioImaging/CMI
- Computational Biology Training
- Electron Microscopy
- Light Microscopy
- Metabolomics
- Next Generation Sequencing
- Preclinical Phenotyping
- Protein Technologies
- Vienna Drosophila Resource Center
- Child Care Center
- VBCF Landing Page
- Projects & Grants
- My VBCF Info-Hub

- Companies at Vienna BioCenter
- Ablevia Biotech
- ACCANIS Biotech
- ADvantage Therapeutics
- Affiris CVD GmbH
- aitiologic GmbH
- BioNTech R&D Austria
- bit.bio discovery GmbH
- CALYXHA Biotechnologies
- G.ST Antivirals
- HeartBeat.bio AG
- HOOKIPA Pharma Inc.
- Miti Biosystems
- MyeloPro Diagnostics and Research GmbH
- Myllia Biotechnology
- Pregenerate
- proxygen GmbH
- QUANTRO Therapeutics
- Thermo Fisher Scientific
- THT Biomaterials GmbH
- Tridem Bioscience
- X4 Pharmaceuticals
- YGION Biomedical GmbH
- LabConsulting
- IG StartUP Solutions GmbH

- Open Positions
- Campus Life
- Life in Vienna

- About Vienna BioCenter
- Who is Here (Members)
- Management Team
- Virtual Tour
- Events at the Vienna BioCenter
- Sustainability
Research Areas
Even though human beings and mammals share many biological traits, the cumulative development of technology, language and culture has led to fundamental changes in our relationships with the environment, other living beings, and ourselves. This has influenced and will continue to influence how human beings have evolved in the ecological niche they have, to a large part, created themselves.

Scientists at the Vienna BioCenter are using bottom-up approaches to discover how individual molecules assemble into sophisticated 3D structures that perform highly specialized cellular functions. By combining biochemistry research, biophysics research, and state-of-the-art structural methods, scientists are resolving macromolecular complexes at atomic resolution, reconstituting multi-component complexes in vitro , studying protein-ligand interactions, and observing molecular machines in action.

To get fundamental insights into how a cell, the basic unit of life, is organized and divides, a highly interactive research community at the Vienna BioCenter uses cutting-edge approaches, including super-resolution microscopy, high-content screening, biochemical reconstitution, and genomics, to functionally dissect the molecular biology of the cell.
Our Cognitive and Behavioral scientists study the neuronal, hormonal, and cognitive bases of behavior. The strength of this research derives from a comparative approach encompassing numerous model organisms and a variety of research approaches that study questions ranging from the development and function of neuronal circuits to animal behavior in social groups. This research investigates the interplay between genetic, physiological, and environmental factors that significantly influence the behavior and cognition of animals and the evolution of behavior.

To understand the vast complexity in biology, many research projects at the Vienna BioCenter have integrated algorithm development, modeling, and high-throughput processing of data. These computational tools enable the next-level analysis of large amounts of biological data, ranging from high-throughput microscopic images to genome sequences or thousands of single-cell transcriptomes, and are also indispensable for evolutionary biology research.

Exploiting the wide range of expertise and model systems at the Vienna BioCenter, researchers ask how certain mutations lead to disease, how organisms defend themselves against pathogens and malignant cells, and how we can use this knowledge to develop better therapeutics and improve agriculture.

Research in gene regulation and gene expression, RNA biology, and epigenetics at the Vienna BioCenter combines diverse approaches ranging from biochemistry and cell biology to genomics and transcriptomics to unravel the connections between genomes and the transcripts they encode. A particular focus is on RNA research, where myriad roles for this nucleic acid have only recently come to light.

Research in Microbiology and Ecosystem Science at Vienna BioCenter aims to reveal structure-function relationships of microbial communities in environmental and medical systems and to reach a comprehensive understanding of host-microbe interactions. Research in this area is essential to understand the role of microbial communities for the functioning of complex ecosystems and their susceptibility to global change due to human activities.

The Vienna BioCenter is highly respected in the Vienna neuroscience scene as well as the global neurobiology community, and uses state-of-the-art techniques to address fundamental questions in the field. How do brains form and evolve? What are the molecular mechanisms that process and store information? How do activity patterns in neural circuits produce perception and behavior? And what mechanisms underlie neurological disorders?

Researchers at Vienna BioCenter discover fundamental molecular mechanisms that operate in a diversity of plants – from unicellular algae to tall, long-lived trees. These mechanisms provide a detailed understanding of the key role plants play in the Earth system. Some of our discoveries may help humanity live within the planet’s natural resource boundaries at a time when the Earth is heating at unprecedented rates.

Scientists at the Vienna BioCenter are using their cutting-edge research infrastructure to uncover the signaling steps and regulatory mechanisms that control tissue, organ, and organismal development. This area of research has enormous biological and medical relevance, providing insights into developmental diseases and cancer, as well as tissue and organ regeneration.
The website uses cookies to ensure you get the best experience on our website.
Analysis / statistics
Anonymous evaluation for troubleshooting and further development
Purpose: error analysis, statistical evaluation of our website accesses, campaign analysis, conversion tracking, retargeting Processing operations: Collection of access data, data from your browser and data about the content accessed; Execution of analysis software and storage of data on your terminal device, anonymization of the data collected; Evaluation of the anonymous data in the form of statistics Storage period: data on your device for up to two years. Joint controller: Google Ireland Limited, Gordon House, Barrow Street, Dublin 4, Ireland Legal basis for data processing: voluntary, consent that can be revoked at any time Consequences of non-consent: No direct impact on the functionality of the website; however limited opportunities for further development and error analysis Data transfer to the USA: Your data is processed by the provider Google in the USA, which involves corresponding risks, e.g. B. a secret data access by US authorities. With your consent, you also consent to the processing of your data in the USA.
An official website of the United States government
Here's how you know
Official websites use .gov A .gov website belongs to an official government organization in the United States.
Secure .gov websites use HTTPS. A lock ( Lock Locked padlock ) or https:// means you've safely connected to the .gov website. Share sensitive information only on official, secure websites.
Our Focus Areas
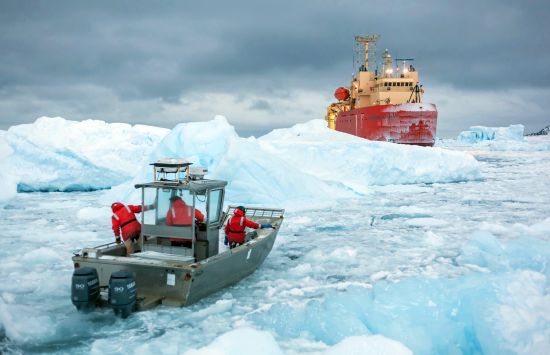
Arctic and Antarctic
Exploring the planet's vulnerable polar regions.
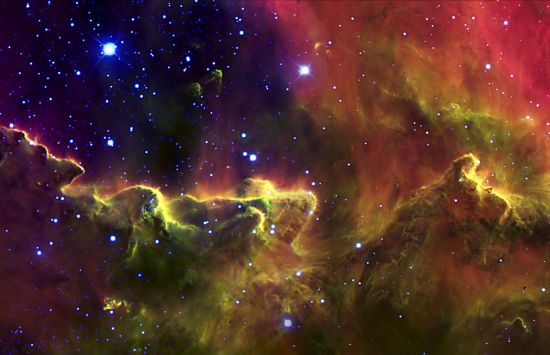
Astronomy and Space
Opening windows to the universe.
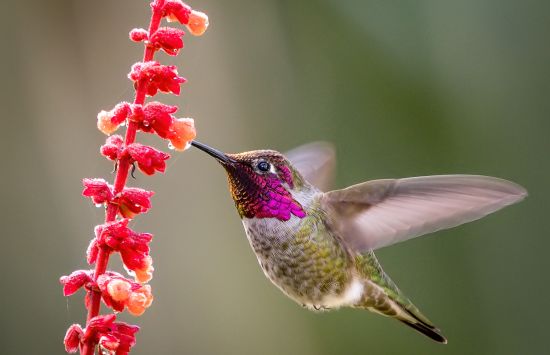
Understanding life in all its forms and sustaining the Earth's ecosystems as they face unprecedented global challenges.
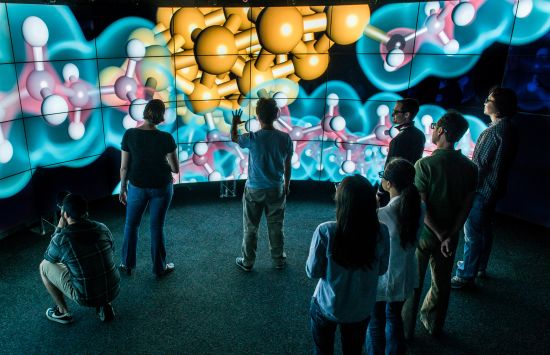
Chemistry and Materials
Understanding the basic properties of matter and forging the chemicals and materials of the future.
Advancing computer and information science and engineering for societal impact.
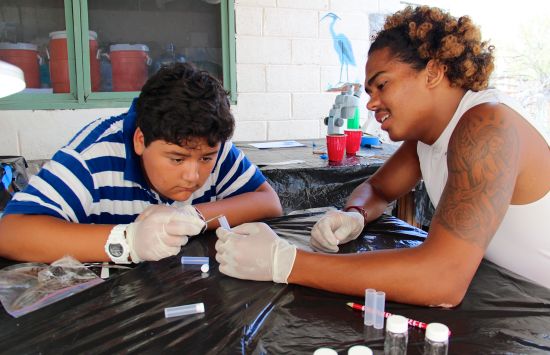
Diversity in STEM
Expanding opportunities in science, technology, engineering and mathematics across the nation.
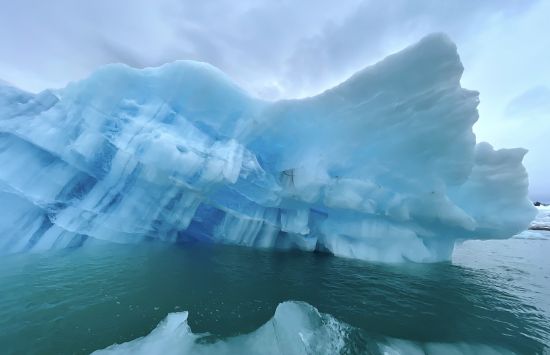
Earth and Environment
Exploring the wonders of our planet to preserve its future.
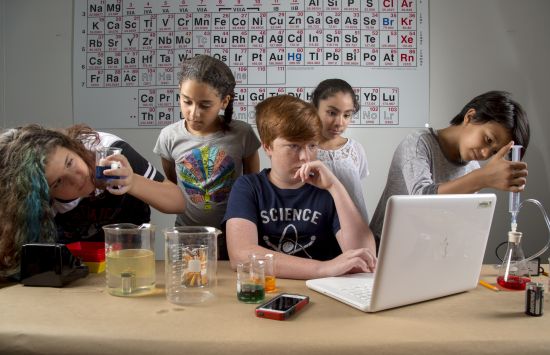
Education and Training
Unleashing the nation's science and engineering talent.
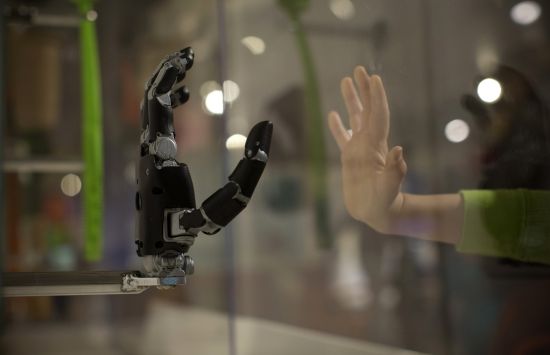
Engineering
Pursuing use-inspired research and innovation to create a future where people can thrive.
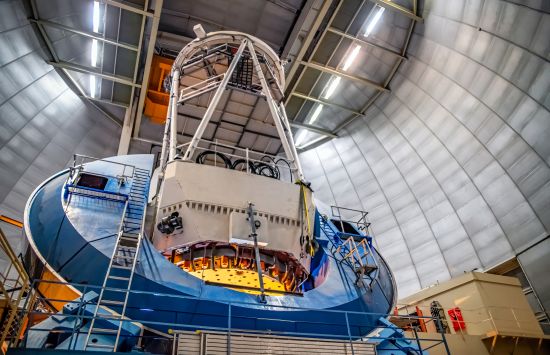
Facilities and Infrastructure
Empowering scientists, engineers and educators to explore, experiment and discover new frontiers.
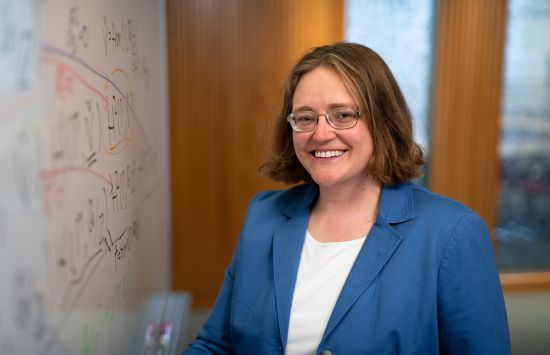
Mathematics
Developing and exploring the language of the universe.
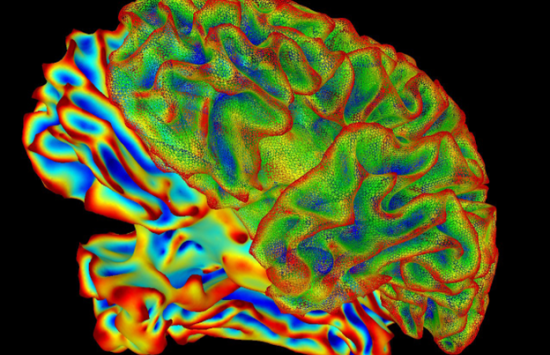
People and Society
Illuminating what makes us human.

Understanding the fundamental workings of the universe — from tiny quantum particles to the largest galaxies.
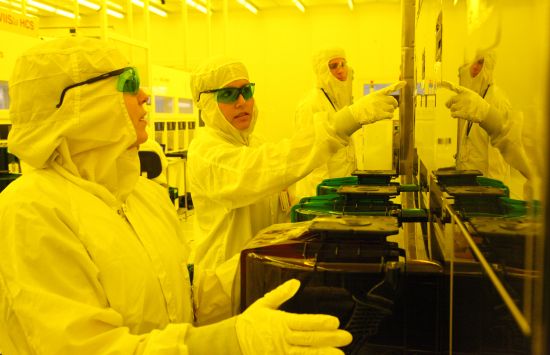
Research Partnerships
Addressing challenges that require collaboration and fresh perspectives.
Interdisciplinary, Convergence and Transformative Research
Research approaches that address societal problems and create new frontiers in science and engineering.
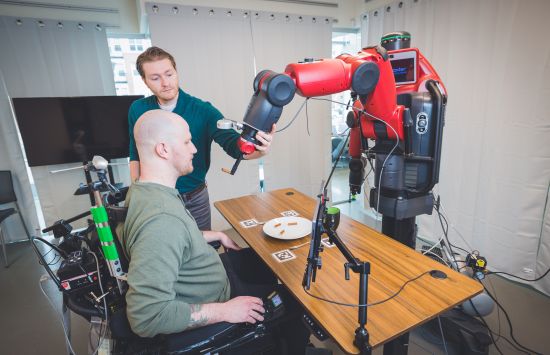
Paving the way for the technologies that will shape the future.
Our key technology areas:
Advanced manufacturing, advanced materials, artificial intelligence, biotechnology, communications and wireless, cyberinfrastructure and advanced computing, cybersecurity, disaster risk and resilience, energy technology, quantum information science and technology, semiconductors and microelectronics.
Developmental Biology
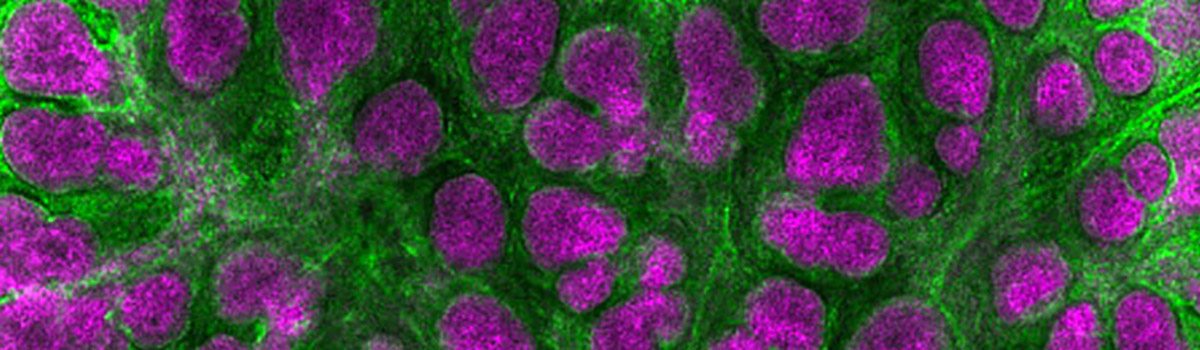
View Principal Investigators in Developmental Biology
Developmental biology aims to understand how an organism develops—how a single cell becomes an organized grouping of cells that is then programmed at specific times to become specialized for certain tasks. Genes control much of an organism’s development, but environmental stimuli also play a role, resulting in the complex “nature vs. nurture” paradigm. Intramural Research Program (IRP) investigators seek a deeper understanding of human development and the many disorders and diseases that can occur when normal development is disrupted.
Developmental biology in the IRP covers an enormous array of scientific investigation:
- Vertebrate embryogenesis : IRP scientists aim to understand embryogenesis at the genomic, cellular, and organism level, often investigating many components within one system—known as developmental systems biology.
- Cellular function and differentiation : Studying how a particular cell evolves to engage a specific function within the body—whether a secretory cell (sweat, tears, insulin), a neuron, a heart muscle cell, or a fat cell—is key to understanding the many diseases associated with malfunction of different cell types.
- Tissue repair : It has long been observed that embryonic development resembles wound healing in many ways—the cellular divisions and adhesions of both are strikingly similar. However, development occurs almost seamlessly, whereas wounds do not heal perfectly. IRP researchers aim to understand the cellular processes behind developmental tissue modulation, which may help define a new era of cellular therapies for damaged adult tissues.
- Cancer : While embryonic development involves a series of highly controlled and coordinated steps, cancer exhibits a lack of cellular control. Understanding the key regulatory pathways behind development may point the way towards therapies designed to modulate disrupted pathways.
- Stem cell research : Stem cells include the pluripotent cells of a developing embryo that evolve to become one of hundreds of different specialized cells as the embryo grows. Stem cells are also found in adult organisms—in bone marrow, blood, and adipose tissue—and IRP researchers work to understand how to isolate and manipulate adult stem cells into becoming specialized cells that could become therapies for a range of diseases, from diabetes to neuromuscular disorders.
- Chromatin and epigenetics : Part of the “nature vs. nurture” paradigm involves non-genetic mechanisms that play a role in switching on and off various genes during development. IRP scientists are at the forefront of research into the importance of chromatin and epigenetics in many aspects of development and disease, including potential uses in gene therapies.
From the genetic basis of development and cell function to biochemical influences, developmental biology research flows through many Institutes and Centers within the IRP. To learn more about the IRP researchers who are affiliated with our developmental biology program, please visit the DevelopmentalBiology@NIH site .
This page was last updated on Tuesday, January 11, 2022
We use cookies to provide you with the best experience and to help improve our website. View Privacy Statement
- About College of Arts & Sciences Message from the Dean Meet the Staff Awards & Recognition News & Events CAScade Magazine Job Opportunities Centers & Research Center for Family and Demographic Research Center for Neuroscience, Mind & Behavior Center for Photochemical Sciences Center for Popular Culture Studies Center of Science and Mathematics Education Institute for the Study of Culture and Society Lake Erie and Watershed Studies National Center for Family & Marriage Research Learning Communities Arts Village Finding Your Voice in Social Justice Global Village La Comunidad Outreach Language Learning Center Psychology Service Center Institute for Psychological Research and Application State Fire School BG Ideas Podcast
- Undergraduate Areas of Study Acting/Directing Actuarial Science Advertising Africana Studies American Culture Studies Applied Mathematics Art Education Art History Asian Studies Biochemistry Biology Chemistry Classical Civilization Communication Computational Data Science Computer Science Creative Writing Data Science Design/Technical Theatre Digital Arts Digital Forensics Ecology and Conservation Biology Economics English Environmental Science Environmental Policy & Analysis Ethnic Studies Film Production Film Studies Fire Administration Forensic Chemistry Forensic Science: Forensic DNA Analysis Forensic Science: Forensic Drug Analysis Forensic Science: Forensic Examination French Geography Geology German Graphic Design History Individualized Planned Program International Studies Journalism Latin Latin American Culture Studies Liberal Studies Marine and Aquatic Biology Mathematics Media Production & Studies Microbiology Music Musical Theatre Neuroscience Paleobiology Philosophy Philosophy, Politics, Economics & Law Physics Popular Culture Political Science Pre-Health Biology Public Relations Psychology Russian Sociology Software Engineering Spanish Statistics Studio Art Theatre Women's, Gender, & Sexuality Studies Youth Theatre Pre-Professional Programs Dentistry Law Medicine Mortuary Science Occupational Therapy Optometry Osteopathy Pharmacy Physician Assistant Veterinary Medicine
- Graduate Doctoral Programs American Cultural Studies Biological Sciences English (Rhetoric and Writing) Mathematics and Statistics Media and Communication Philosophy - Applied Photochemical Sciences Psychology Sociology Theatre Masters Programs American Culture Studies Art Art History Biological Sciences Chemistry Computer Science Creative Writing English (online) English Teaching European Studies Forensic Science French Geology Geospatial Sciences - Applied German Masters Programs History Literature & Textual Studies (English) Mathematics and Statistics Media and Communication Philosophy Physics Popular Culture Sociology Professional Writing & Rhetoric Public Administration Spanish Strategic Communication (Online) Theatre Graduate Certificates Ethnic Studies Geospatial Technology International/Intercultural Communication International Scientific and Technical Communications Performance Studies Public History Social & Interactive Media Spanish TESOL Women's Studies
- Academic Units Departments Africana Studies American Culture Studies Asian Studies Biological Sciences Chemistry Computer Science English Ethnic Studies History International Studies Mathematics & Statistics Philosophy Physics & Astronomy Political Science Popular Culture Psychology Sociology Theatre & Film Women's, Gender, & Sexuality Studies World Languages and Cultures Schools School of Art School of Cultural & Critical Studies School of Earth, Environment & Society School of Media & Communication Learning Communities Arts Village Finding Your Voice in Social Justice Global Village La Comunidad
- Students Current Students Bachelor of Liberal Studies Degree Audit Reporting System Faculty Mentors Find Your Advisor GPA Calculator Helpful Links Honors College Internships/Co-ops Learning Communities Majors and Checksheets Pre-Professional Programs Scholarships Transfer Credit Evaluation Undergraduate Student Handbook Future Students Advanced Placement Apply Now Degree Audit Reporting System Helpful Links Honors College Learning Communities Majors and Checksheets Pre-Professional Programs Scholarships University Orientation Misc. Links Kuhlin Hub for Career Design and Connections Education Abroad MyBGSU Parent and Family Resources Residence Life Student Financial Aid " role="menuitem" tabindex="-1">Searchable Scholarship Guide and Application
- Faculty/Staff Chairs & Directors Handbook Job Openings in the College Schedule Development Packet College Forms Software Site License Agreements Staff Links Human Resources Administrative Staff Council Classified Staff Council Arts & Sciences Committees Arts & Sciences Council Arts and Sciences Staff Council Council of Chairs & Directors Curriculum Committees Distinguished Teaching Award Committee Diversity and Inclusion Committee Promotion and Tenure Review Committee University Committees Faculty Senate Representatives Graduate Council Undergraduate Council Representatives University-Level Review Committee
- Alumni Accomplished Graduates Alumni Connections Transformations Magazine
Research Areas
The Program in Biochemistry and Molecular Biology consists of research faculty working in three main areas of research; environmental microbiology, plant biology, & cancer biology.
The department has traditionally maintained a strength in the area of microbiology in order to support an undergraduate microbiology major. The undergraduate major has yielded a curriculum that serves as an intellectual foundation for a large segment of the biochemistry faculty. Whereas the Biochemistry Group shares a common theme of protein structure and function, a large number of the research-active faculty examine proteins that mediate key cell functions of bacteria and parasites.
Microbiology: The department has traditionally maintained a strength in the area of microbiology in order to support an undergraduate microbiology major. The undergraduate major has yielded a curriculum that serves as an intellectual foundation for a large segment of the biochemistry faculty. Whereas the Biochemistry Group shares a common theme of protein structure and function, a large number of the research-active faculty examine proteins that mediate key cell functions of bacteria and parasites. In addition, the support facilities for microbiology and parasitology research and training are readily available (e. g. Animal Research Facility, Electron Microscopy Facility, Microbiology Prep Room). Thus, we have established an environment in which microbiologists can interact and quickly develop their careers. Given recent federal and private funding interests in microbial genomics, microbial diversity, antibiotic resistance, bioremediation, extremophiles and host/pathogen interactions, it makes good sense to continue to hire microbiologists and parasitologists in the future. Thus, a secondary underlying theme in building and maintaining an active group of biochemists is to identify research interactions within the realm of molecular microbiology and parasitology.
Structure, Function and Dynamics of Biomolecules: We are a group of biochemists engaged in different aspects of protein structure and function to understand important biological processes at the molecular level. Indeed it is an exciting time for biochemists in general, because the recent explosion of genomic data has identified novel genes whose products must be characterized with respect to their structure, function and physical association with other cellular components. The current research topics being addressed here at BGSU all utilize typical preparative and analytical tools including site-directed mutagenesis, HPLC for rapid high-yield protein purification, analytical ultracentrifugation, absorption spectroscopy of redox centers, and structural determination by NMR. In collaboration with the biologically-oriented chemists in the Chemistry Department, we are developing a new research unit, the Center for Biomolecular Sciences, to foster collaborative research activities between faculty and students in both departments.
Department of Biological Sciences
419-372-2332 [email protected]
Dr. Dan Pavuk, Chair
Department of Biological Sciences Bowling Green State University Bowling Green, OH 43403 419-372-2333 [email protected]
Michele Nagel, Office Manager
419-372-2332 [email protected]
Ray Larsen, Grad Coordinator
419-372-9559 [email protected]
Updated: 07/01/2019 09:50AM
Center for Structural Biology
Iverson lab provides new insights on chemotaxis.
Posted by daviskd2 on Friday, May 3, 2024 in News .
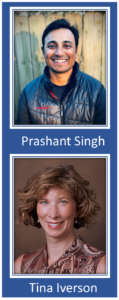
Chemotaxis is a key characteristic shared by all infectious bacteria. It’s a versatile process that allows bacteria to swim toward energy-rich molecules, find preferred niches for infection, avoid harmful species, change speeds and fully stop to form biofilms. Chemotaxis is also essential for virulence in animals and a potential target for new therapeutics. But first, the process itself needs to be better understood.
The Iverson lab is now working to identify how an expanded range of different protein partners bind to the flagellar motor during chemotaxis, and hope that this will lead to ways to disrupt chemotaxis during infection.
Read the full article on the Basic Sciences website .
Leave a Response
You must be logged in to post a comment
Your Vanderbilt
- Current Students
- Faculty & Staff
- International Students
- Parents & Family
- Prospective Students
- Researchers
- Sports Fans
- Visitors & Neighbors
Follow me on Twitter
Quick links.
- PeopleFinder
You must be logged in to post a comment.
Health Science Center
What Can we help you find?
Popular Searches
- Academic Calendar
- Study Abroad
- Majors & Minors
- Request Info
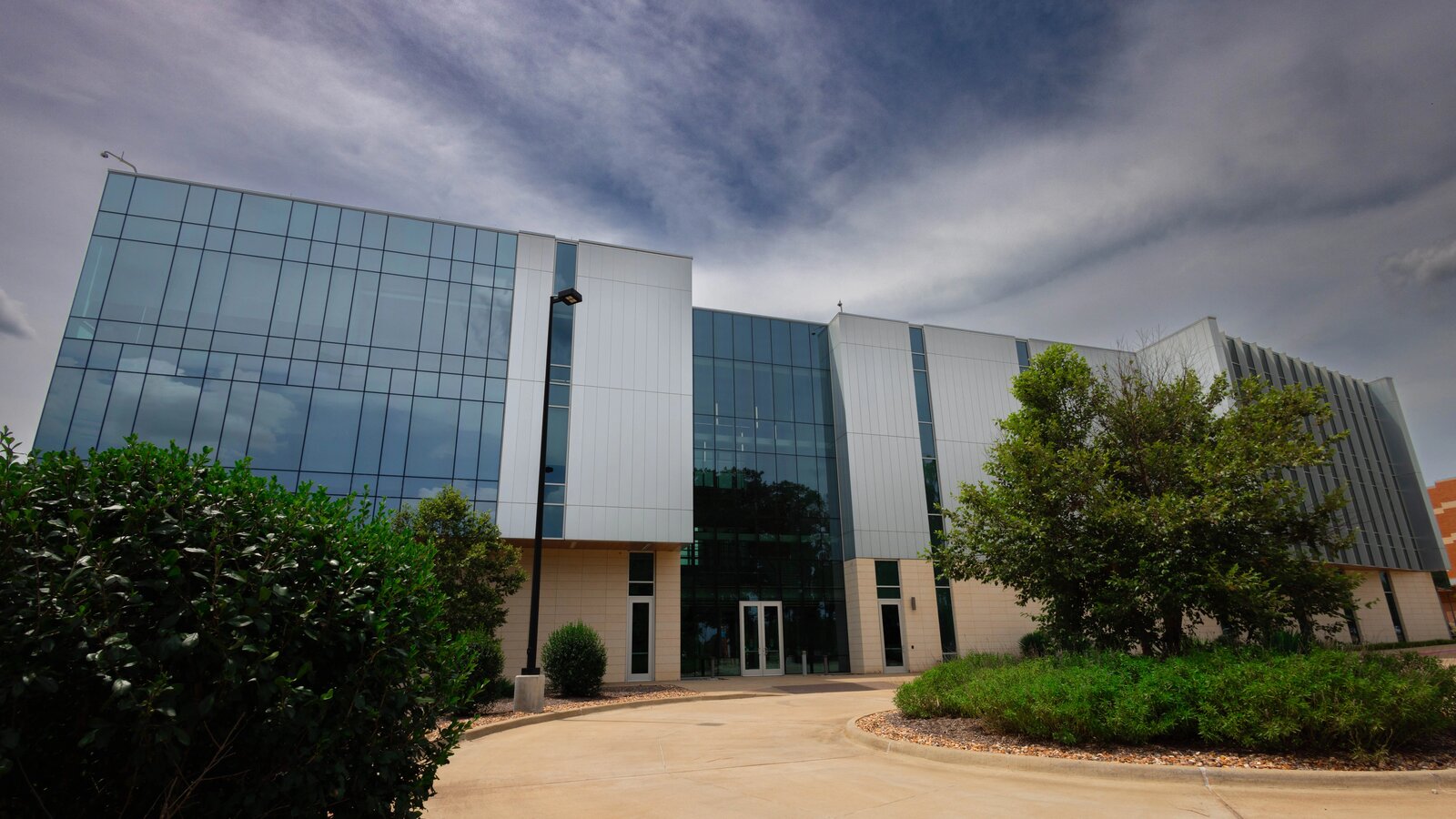
UT Tyler Health Science Center
Build a healthier tomorrow.
Home to the region’s only academic medical center, The University of Texas at Tyler Health Science Center is one of the five campuses of UT Tyler. Two of UT Tyler’s four health-related schools have a presence on this campus: the School of Health Professions and the School of Medicine.
Campus History
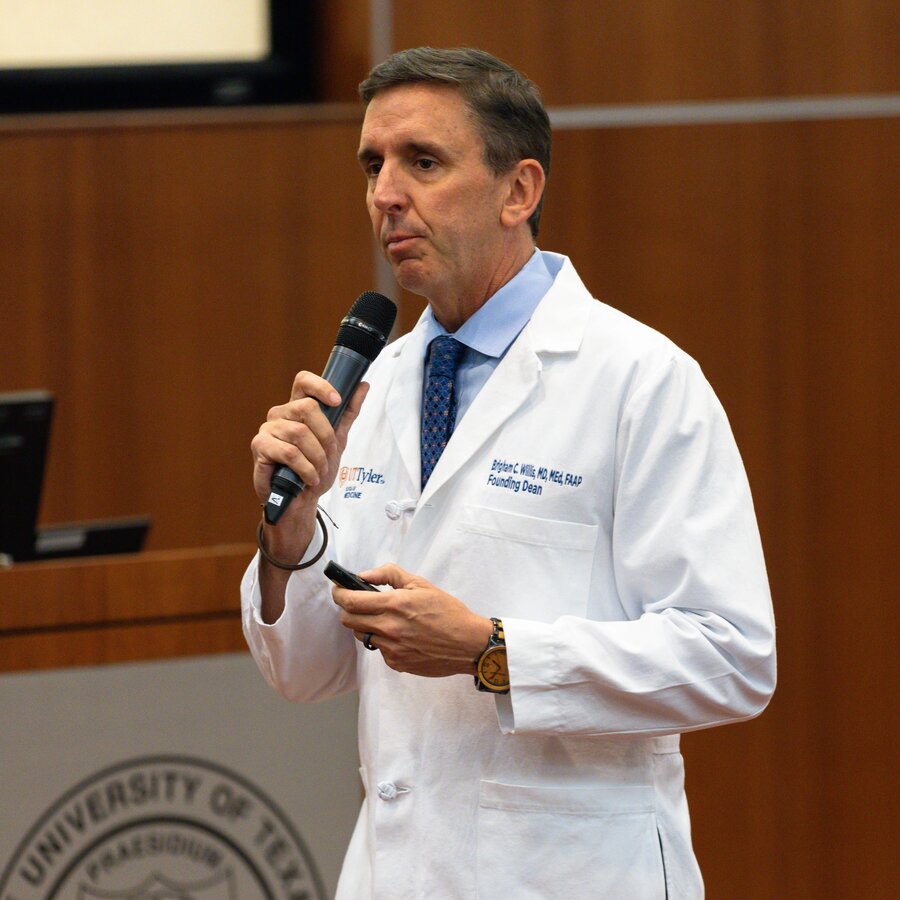
Our Beginnings
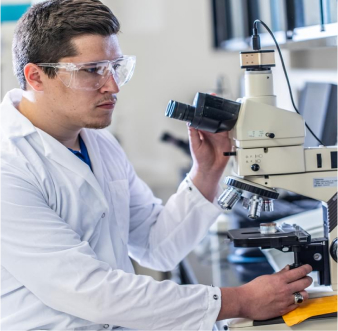
Joining the University of Texas System
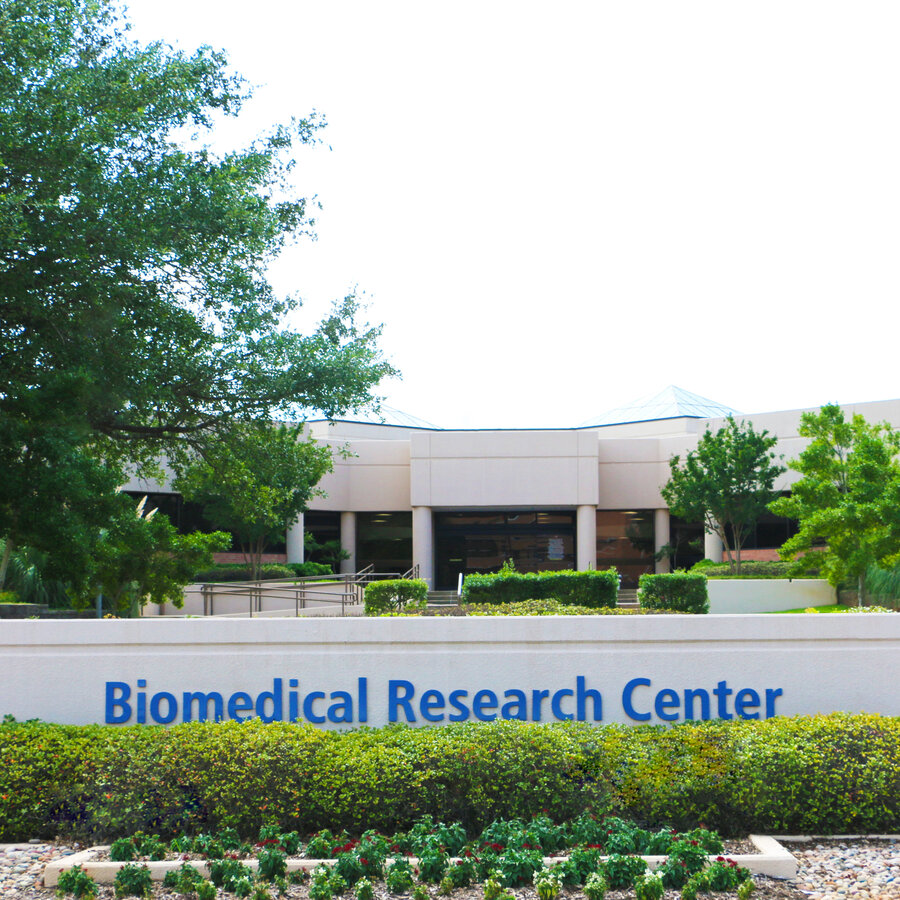
A Name Change
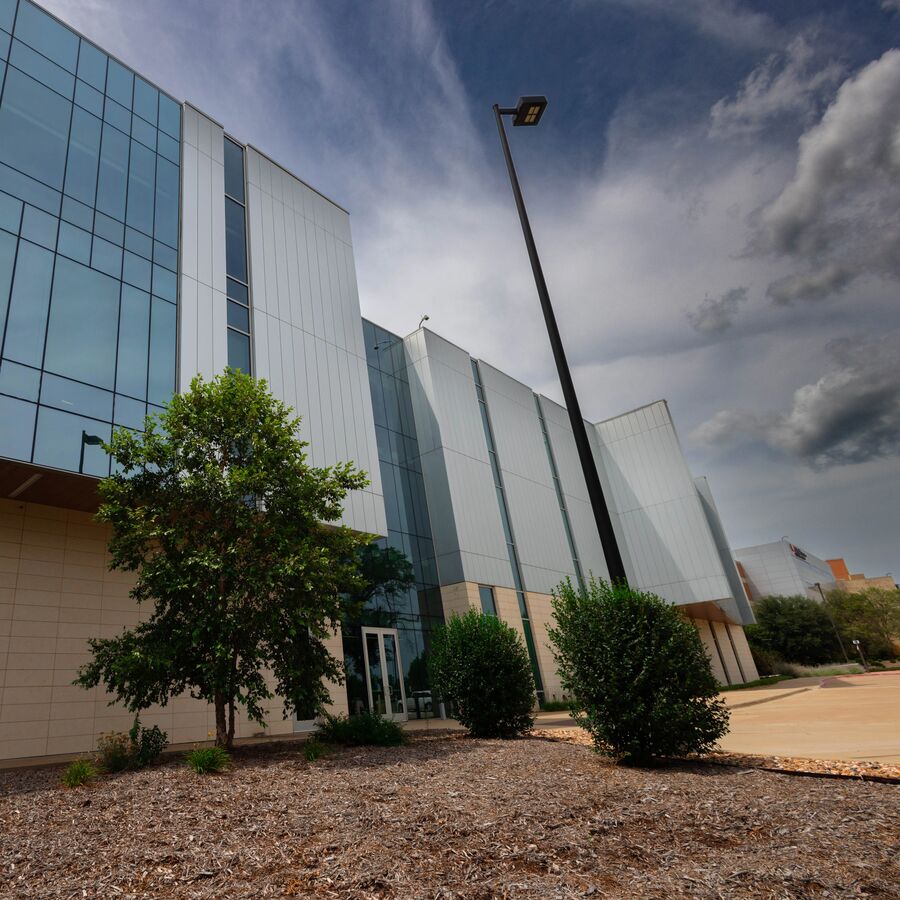
New Programs
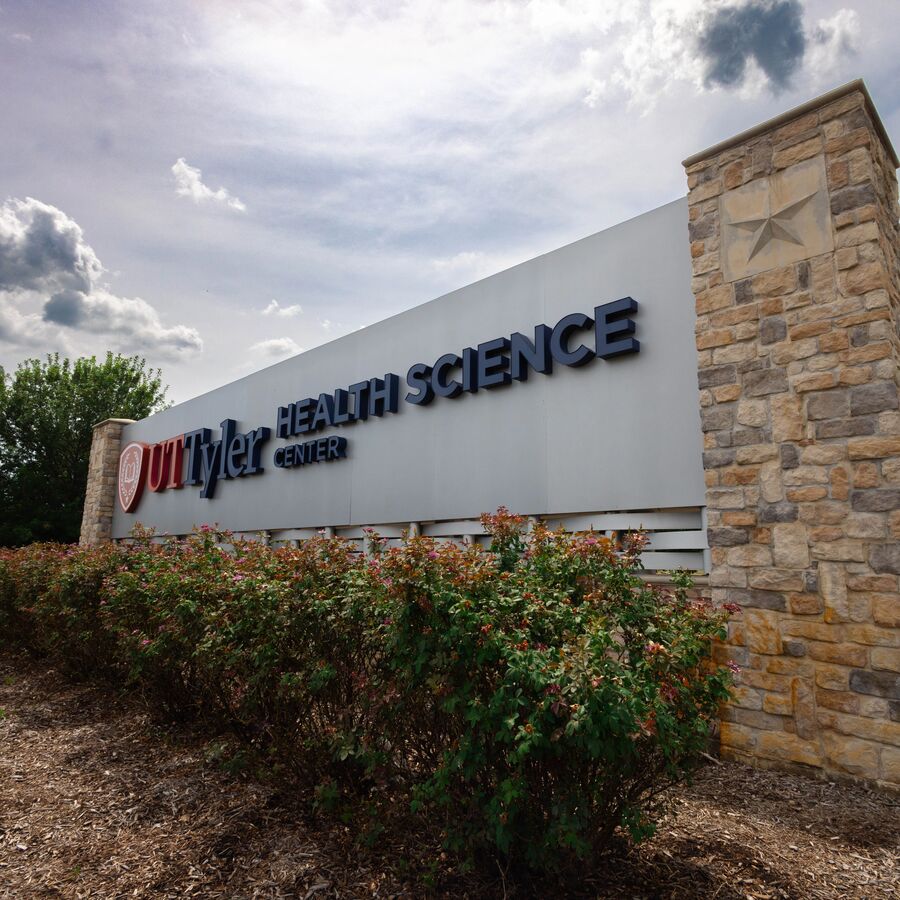
Campus Programs and Facilities
The UT Tyler Health Science Center facility offers an array of crucial medical and healthcare education resources, fostering an environment dedicated to excellence in education. From cutting-edge simulation labs to dedicated research spaces, every aspect of the UT Tyler Health Science Center is designed to enhance the educational experience. This dynamic campus is not just a hub for learning; it’s a catalyst for progress in healthcare education and a testament to UT Tyler's commitment to shaping the future of healthcare in the East Texas region.
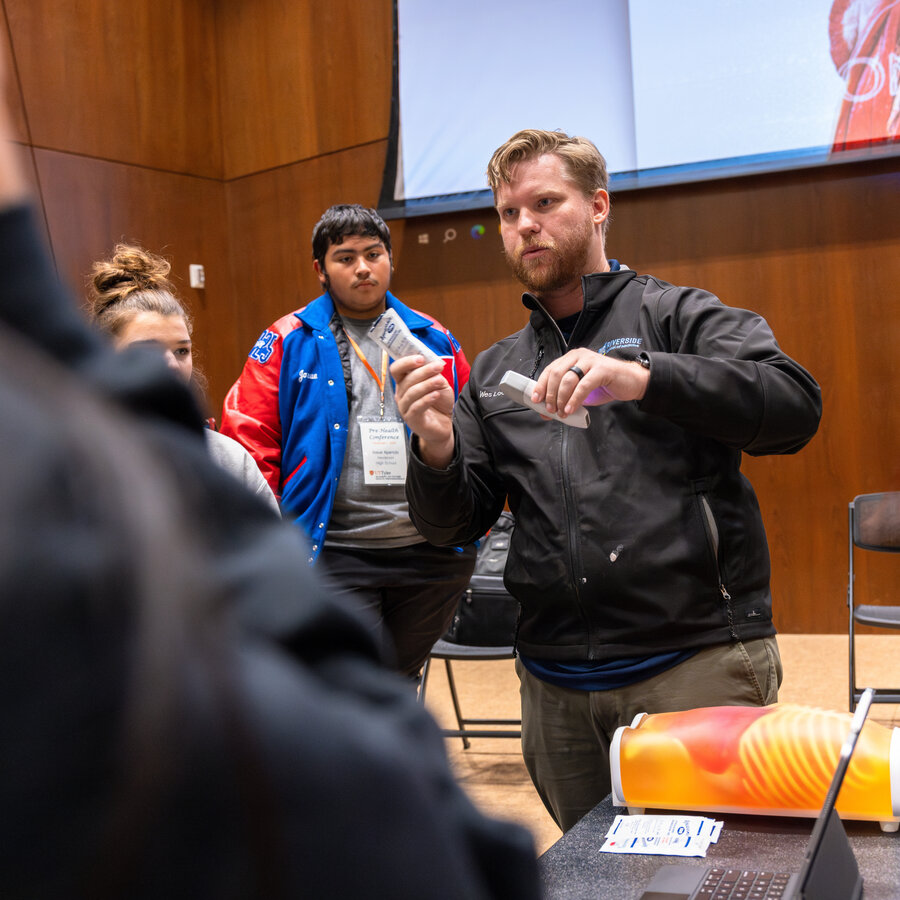
Office of Health Affairs
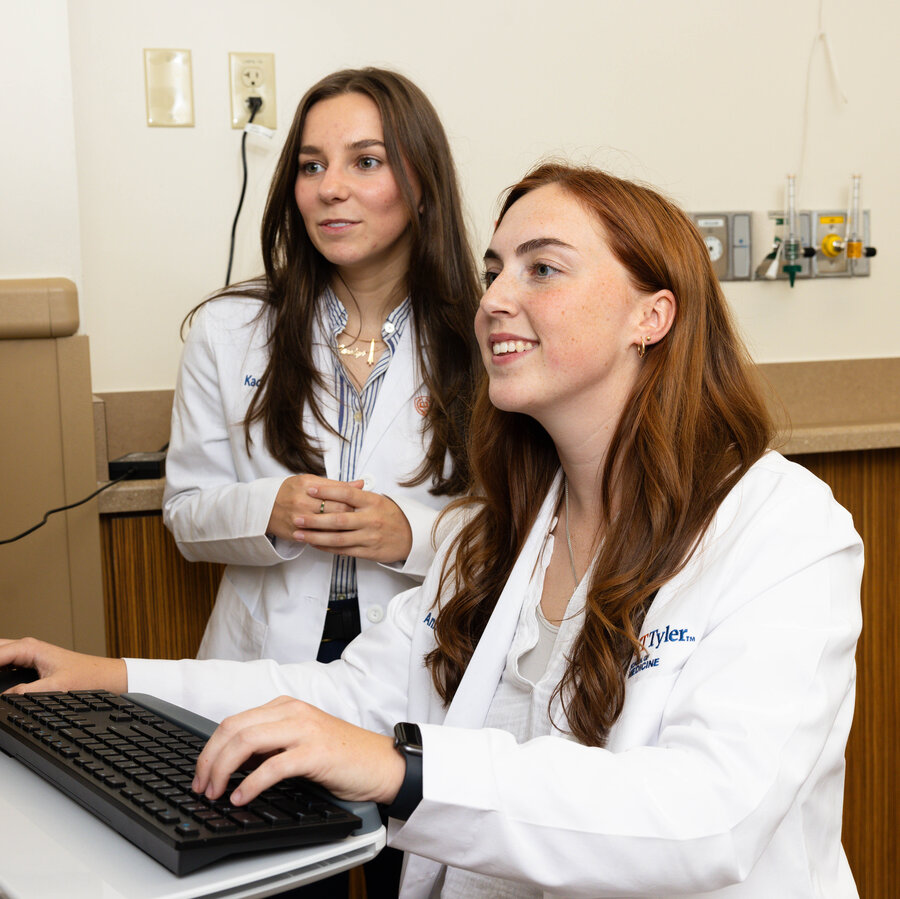
School of Health Professions
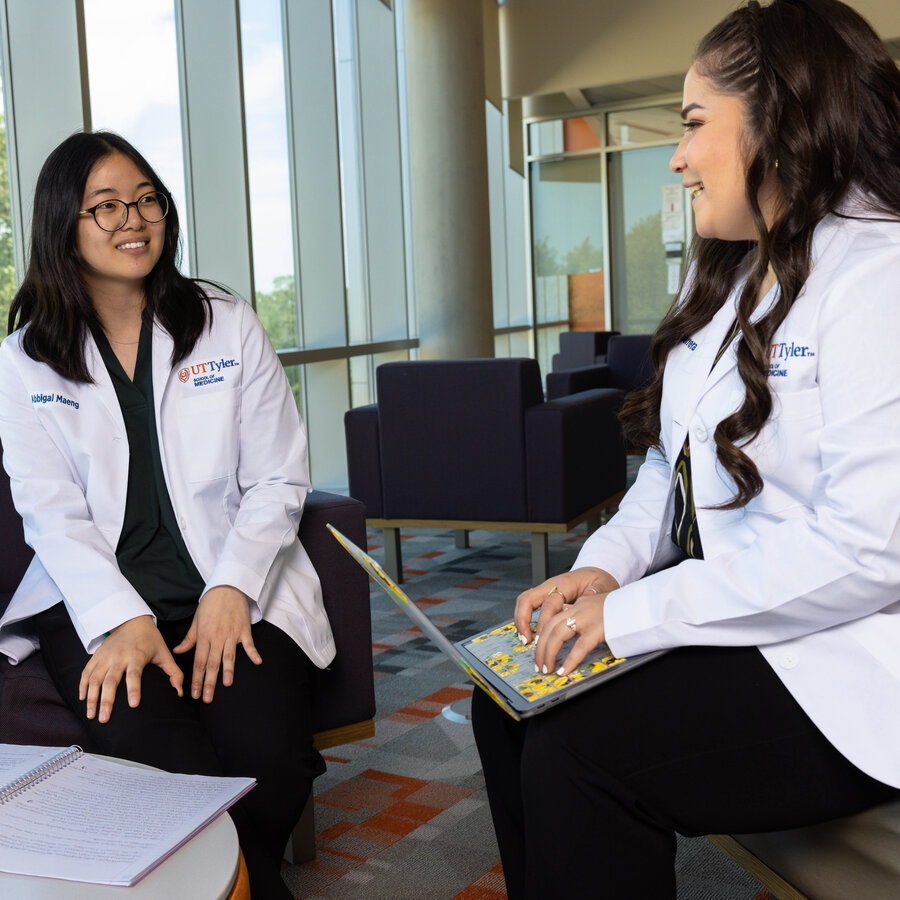
School of Medicine
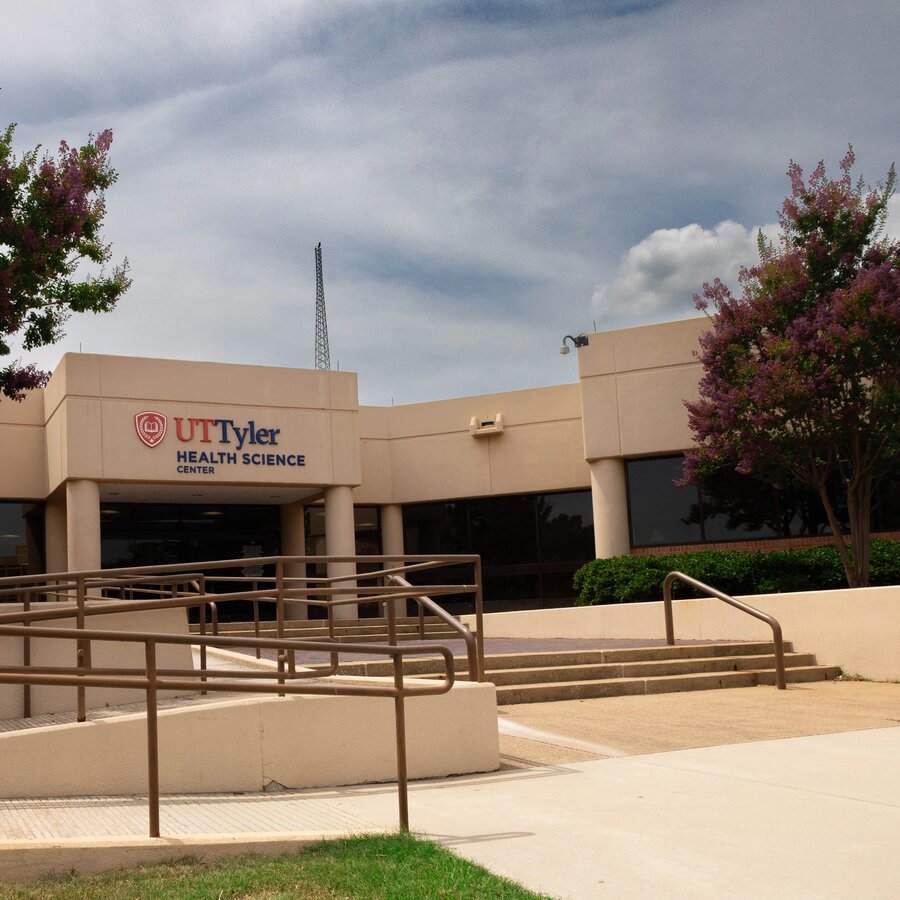
Center for Biomedical Research
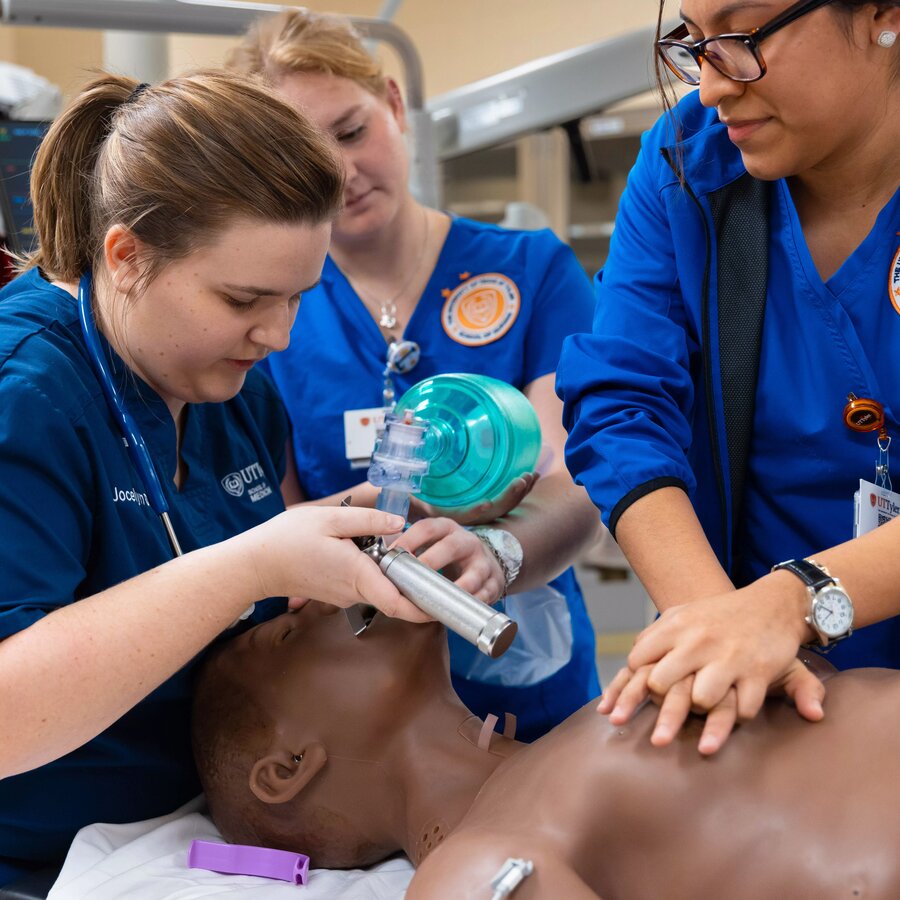
Simulation in Medicine and Immersive Learning Experience Center
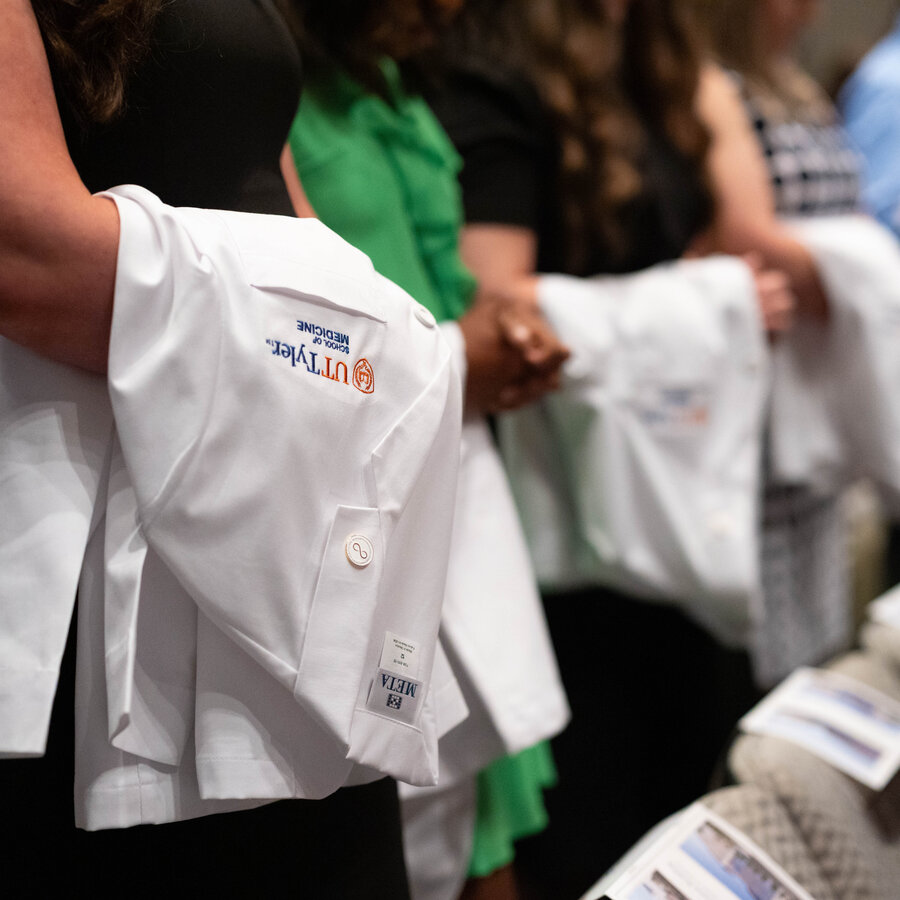
Watson W. Wise Medical Research Library
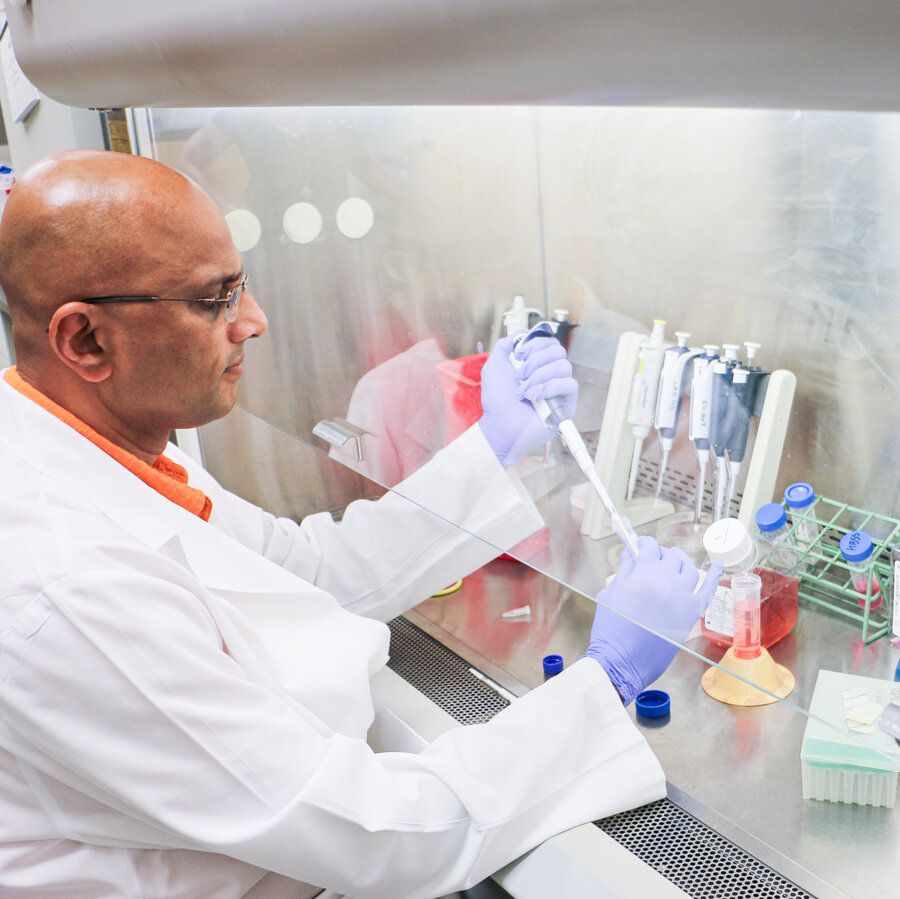
Public Health Laboratory of East Texas
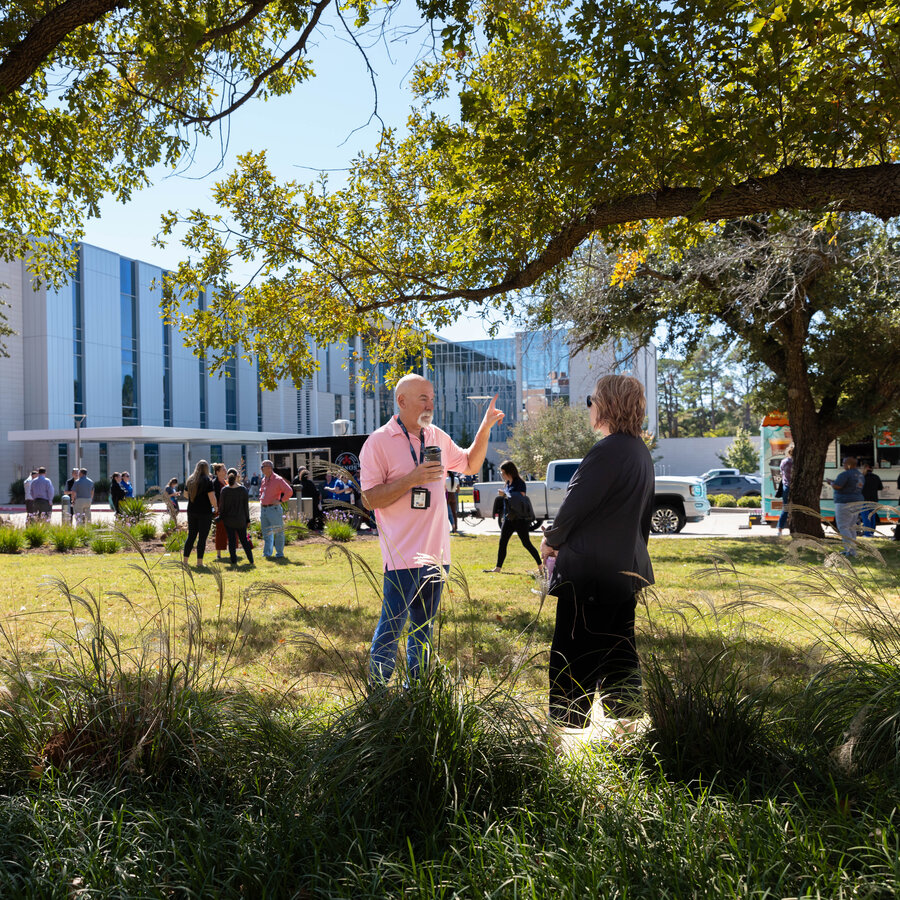
UT Health North Campus Tyler (UTHET)
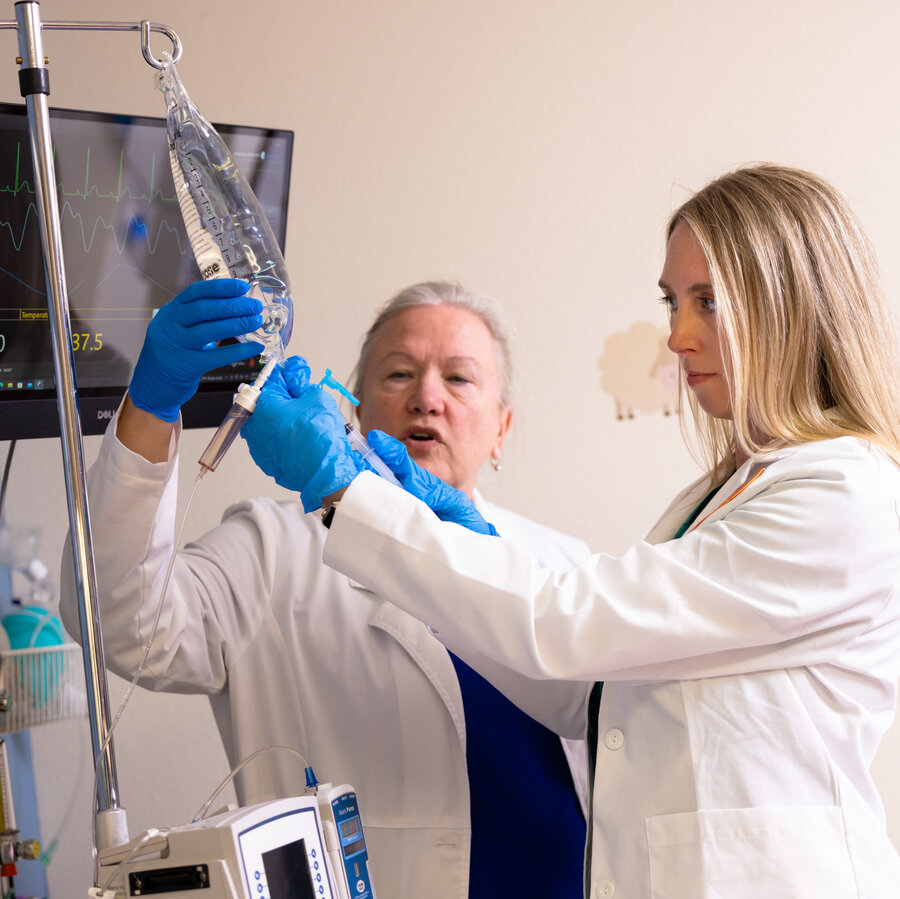
HOPE Cancer Center
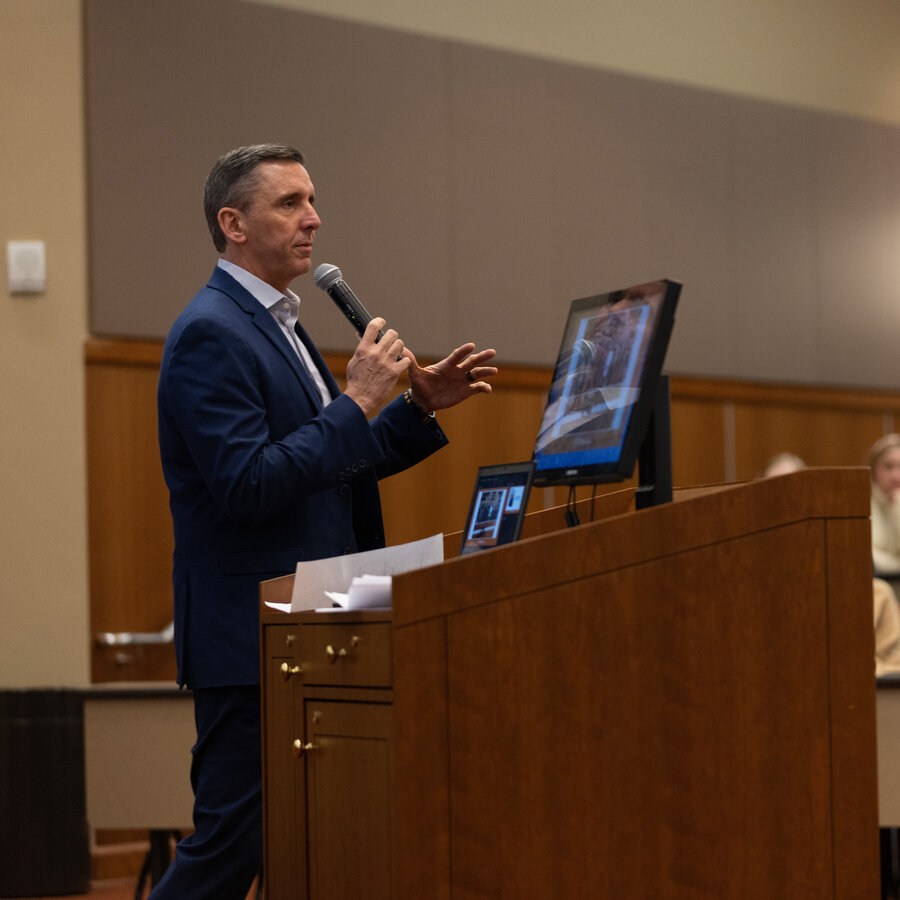
A Regional Leader in Health Research
UT Tyler pioneers solutions to improve health. Several research centers, including the Center for Mycobacterial Treatment and Discovery and the Center for Biomedical Research, are housed on this campus. The centers build on our history of innovative treatments for lung disease and focus on the health concerns of rural populations through projects funded by agencies like the National Institutes of Health and the Centers for Disease Control and Prevention. Students benefit from hands-on research opportunities and instruction informed by the latest developments in the field.
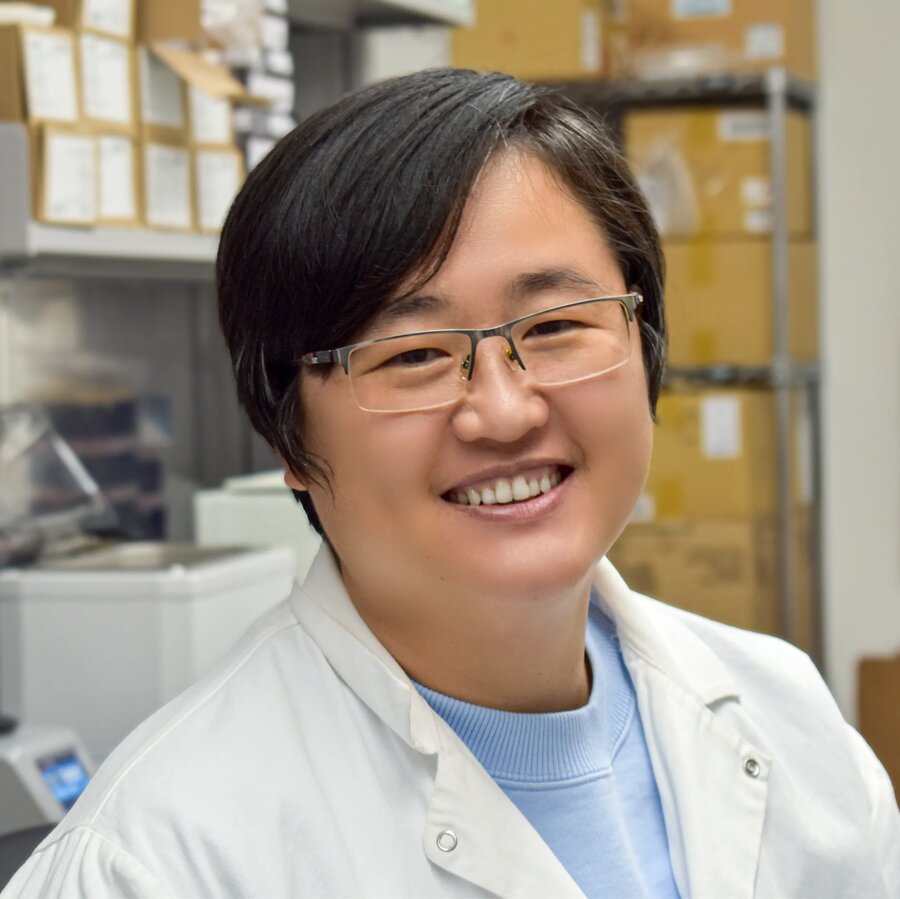
Dr. Maolin Lu
Assistant Professor of Cellular and Molecular Biology
Meet Professor Lu
Community Outreach and Engagement
Ut health east texas.
In its regional network of hospitals, clinics and other facilities, UT Health East Texas delivers world-class care to thousands of patients each year while conducting clinical trials and training the next generation of professionals through UT Tyler’s unique programs. The UT Tyler Health Science Center is home to UT Health North Campus Tyler .
Public Health Programs
Faculty, staff and students at the UT Tyler Health Science Center campus connect their expertise with local community needs to assist traditionally underserved populations through an array of health and outreach programs, including behavioral health telemedicine services for rural populations, cancer screenings, parental education, lifestyle changes and more.
Regional Health Resources
To strengthen regional healthcare, we train community health workers, promote healthcare careers in underrepresented communities and support community health education and development efforts.
Connect With Us
The university of texas at tyler health science center.
Phone: 903.877.7777
We’re pioneering the future of healthcare in East Texas. Find out how you can join us.
11937 U.S. Hwy. 271 Tyler, TX 75708-3154
Institute of Politics
- Internships & Careers
- Internship Hub
Project Unloaded Community Engagement Intern
- Location Chicago, IL Modality Leaning In-Person Classification Both Organization Project Unloaded
- Nonprofit/Advocacy
Overview of Organization: The mission of Project Unloaded is to create a new cultural narrative that guns make us less safe. Research shows that teens and young adults are forming opinions and making decisions about guns. Through creative and cultural campaigns, Project Unloaded establishes safe spaces for open conversations about guns and provides accurate information about gun ownership to inspire the next generation to choose on their own terms not to own or use guns. Internship Summary: Project Unloaded is excited to hire a Community Engagement Intern for Summer 2024. The intern will further Project Unloaded’s Community Partner Program by supporting the creation of a toolkit of resources for teens and youth organizations interested in reducing gun violence by changing cultural narratives around gun use. In addition, this intern will also support implementation of Project Unloaded’s summer 2024 programs in Chicago and Sacramento. Eligible applicants will be incoming and current college or graduate students. Responsibilities: - Support the creation of a toolkit for teens and teen-serving organizations interested in addressing gun violence through narrative and culture change - Research potential community partnerships in Project Unloaded’s key areas of focus - Manage relevant partnerships for toolkit creation - Provide technical support for community-based organizations implementing Project Unloaded programming - Support organizations and participants in the Project Unloaded summer program(s) with their day-to day curriculum completion - Perform other duties as assigned Qualifications: - Strong communication skills - Self directed and good at taking initiative - Passionate about stopping gun violence Location, Modality: This internship leans in person and will take play in Chicago Start and End Date: The internship will run from June 10 to September 27, with the flexibility to accommodate specific scheduling needs.
We primarily work remotely however, this intern will support our Community Partner work through on site visits to the program during the week and meetings at our organizations's shared meeting space. Specific days will be finalized based on the intern's schedule.
- Apply For This Internship
Have Questions?
Stay informed.
Our emails are the best way to keep up-to-date on all of our events and programming.

IMAGES
VIDEO
COMMENTS
Research Areas. Research activity in the Biology Department spans the full range of biological organization, from molecules to ecosystems. Main research fields are indicated here, as links to groups of faculty doing research in those areas.
Areas of Research. For over 50 years, we have played a central role in the growth of molecular life sciences and the revolution in molecular and cellular biology, genetics, genomics, and computational biology.
The life sciences embrace a great array of intellectual activity, a continuum extending from the search for the origin of life and the detailed structure of the macromolecules that make life possible to understanding of the total ecology of planet Earth. The millions of micro-organisms and plant and animal species interacting in the air, the soil, freshwater ponds and streams, and the oceans ...
Biological sciences encompasses all the divisions of natural sciences examining various aspects of vital processes. The concept includes anatomy, physiology, cell biology, biochemistry and ...
Areas of focus include cell biology, population and molecular genetics, epigenetics, microbiology, virology, immunology, structural biology, animal/plant pathology, genomics, bioinformatics, and molecular evolution. Interactive clusters of research groups focus on plant and animal virology, microbial physiology and cell biology, comparative ...
Research Areas. Biochemistry, Biophysics & Structural Biology. Biochemistry and Biophysics are the foundation of all cellular processes and systems. Biochemical processes account for the functions of cellular building blocks, from nucleic acids and proteins to lipids and metabolites, and the formation of complex networks that make a cell or ...
It is also important to know if the research area has sufficient unanswered questions that will be interesting to funding agencies. Try to understand if the field has long-term potential. Scientists often work on certain research areas for decades and so thinking ahead about hypothetical questions and probable answers is one key to success.
Key Research Areas. Research at the Faculty of Life Sciences focus on the fundamental understanding of biological systems, their organisation and evolution. Curiosity-driven basic research in Biology, Pharmacy and Nutritional Sciences provides the basis for application-oriented research to find sustainable solutions to global challenges and ...
Ecology and Evolution. Heredity, Development and Disease. Integrative Membrane Biology. Microbiology. Neuroscience. Plant Sciences. Sport and Exercise Sciences. Structural Biology. Listen to some of our current research students and leading academics talking about some of our key research areas and what projects they are working on that match ...
Research Areas. Making sustained progress against cancer requires advances across the research continuum, from the biology of cancer cells to studies of large populations. Learn about the breadth of NCI's work and the programs the institute supports to advance cancer research.
Our researchers work in the areas of sensory neurobiology (especially vision and olfaction), stress and pain processes, circadian rhythms, control of social behaviors, and computational neuroscience. We collaborate with colleagues in the departments of chemistry and biochemistry, psychology and anthropology, among others. Advancing human and ...
Indeed, research as a whole flourishes with a diverse range of group sizes, with large groups expanding and building up research, and small groups starting new areas of inquiry . Whatever the size, institutes work best when grouped around either a technology (e.g., the Structural Studies Division at the LMB) or around a biological topic (e.g ...
The Cancer Systems Biology Consortium (CSBC), which includes cancer biologists, engineers, mathematicians, physicists, and oncologists, aims to tackle the most perplexing issues in cancer to increase our understanding of tumor biology, treatment options, and patient outcomes.The initiative takes an integrative approach to cancer research, with the goal of improving the lives of people with cancer.
Focus Areas. The mission of the Department of Cancer Biology is to identify and understand the causes of cancer, to develop innovative approaches to reduce cancer incidence, to create and test novel and more effective therapies, and to translate these findings into clinical care for the benefit of patients. Research in our department is highly ...
Area of Biomedical Science Evaluated. Within the broad area of microbiology, this paper is focused exclusively on the outstanding scientific opportunities for Harvard Medical School, and Harvard University as a whole, in the area of microbial chemical ecology and its various impacts on human health. Since its inception late in the 19th century ...
Core Research Areas. There are many ways to focus your studies or research in marine biology, and it can be difficult to know where to start. The University of Washington has faculty with expertise in a diverse range of research areas related to marine biology. To help you explore these areas, we group many topics of interest into four 'core ...
Stem Cells, Development, Regeneration. Scientists at the Vienna BioCenter are using their cutting-edge research infrastructure to uncover the signaling steps and regulatory mechanisms that control tissue, organ, and organismal development. This area of research has enormous biological and medical relevance, providing insights into developmental ...
Cell biological research in the Intramural Research Program (IRP) reflects this spectrum, with concentrated expertise in six key areas that span multiple biological systems: Apoptosis - In every healthy organism, cells die through a carefully regulated process of programmed cell death, known as apoptosis. Apoptosis is common to many ...
Our Focus Areas. Arctic and Antarctic ... Exploring the planet's vulnerable polar regions. Astronomy and Space. Opening windows to the universe. Biology. Understanding life in all its forms and sustaining the Earth's ecosystems as they face unprecedented global challenges. ... Pursuing use-inspired research and innovation to create a future ...
Understanding the key regulatory pathways behind development may point the way towards therapies designed to modulate disrupted pathways. Stem cell research: Stem cells include the pluripotent cells of a developing embryo that evolve to become one of hundreds of different specialized cells as the embryo grows. Stem cells are also found in adult ...
Key Biodiversity Areas (KBAs) are the most important places in the world for species and their habitats. Faced with a global environmental crisis we need to focus our collective efforts on conserving the places that matter most. The KBA Programme supports the identification, mapping, monitoring and conservation of KBAs to help safeguard the ...
Research Areas. The Program in Biochemistry and Molecular Biology consists of research faculty working in three main areas of research; environmental microbiology, plant biology, & cancer biology. The department has traditionally maintained a strength in the area of microbiology in order to support an undergraduate microbiology major.
Marine biology is a key area for high school research, especially looking into coral bleaching and its impact on ocean life. Coral reefs are crucial for marine ecosystems, acting as homes and food sources for many sea creatures. ... Starting a biology research project is an exciting step into discovering new insights about the natural world ...
The lab of Tina Iverson, Louise B. McGavock Professor and professor of pharmacology, in collaboration with researchers at the University of California, San Francisco; Stanford University; and The Weizmann Institute of Science in Israel have published new work in Nature Microbiology, providing new insights on chemotaxis. Chemotaxis is a key characteristic shared by all infectious...
Pioneering research, innovating academic programs and impacting our community. ... The library features both private and public study areas and maintains a collection of over 5,000 periodicals and 3,000 books, most of which are easily accessible in an electronic format. ... Assistant Professor of Cellular and Molecular Biology. Meet Professor Lu.
Research shows that teens and young adults are forming opinions and making decisions about guns. Through creative and cultural campaigns, Project Unloaded establishes safe spaces for open conversations about guns and provides accurate information about gun ownership to inspire the next generation to choose on their own terms not to own or use guns.