Click through the PLOS taxonomy to find articles in your field.
For more information about PLOS Subject Areas, click here .
Loading metrics
Open Access
Peer-reviewed
Research Article

Neurophysiological Effects of Sleep Deprivation in Healthy Adults, a Pilot Study
* E-mail: [email protected]
Affiliations Department of Psychiatry, VU University Medical Center, Amsterdam, The Netherlands, Neuroscience Campus Amsterdam, VU University Medical Center, Amsterdam, The Netherlands
Affiliation Neuroimaging Center University Medical Center, Groningen, The Netherlands
Affiliations Department of Nuclear Medicine & PET Research, VU University Medical Center, Amsterdam, The Netherlands, Neuroscience Campus Amsterdam, VU University Medical Center, Amsterdam, The Netherlands
Current address: Department of Psychiatry, Erasmus Medical Center, Rotterdam, The Netherlands
- Ursula M. H. Klumpers,
- Dick J. Veltman,
- Marie-Jose van Tol,
- Reina W. Kloet,
- Ronald Boellaard,
- Adriaan A. Lammertsma,
- Witte J. G. Hoogendijk
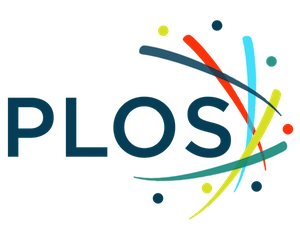
- Published: January 21, 2015
- https://doi.org/10.1371/journal.pone.0116906
- Reader Comments
Total sleep deprivation (TSD) may induce fatigue, neurocognitive slowing and mood changes, which are partly compensated by stress regulating brain systems, resulting in altered dopamine and cortisol levels in order to stay awake if needed. These systems, however, have never been studied in concert. At baseline, after a regular night of sleep, and the next morning after TSD, 12 healthy subjects performed a semantic affective classification functional magnetic resonance imaging (fMRI) task, followed by a [ 11 C]raclopride positron emission tomography (PET) scan. Saliva cortisol levels were acquired at 7 time points during both days. Affective symptoms were measured using Beck Depression Inventory (BDI), Spielberger State Trait Anxiety Index (STAI) and visual analogue scales. After TSD, perceived energy levels, concentration, and speed of thought decreased significantly, whereas mood did not. During fMRI, response speed decreased for neutral words and positive targets, and accuracy decreased trendwise for neutral words and for positive targets with a negative distracter. Following TSD, processing of positive words was associated with increased left dorsolateral prefrontal activation. Processing of emotional words in general was associated with increased insular activity, whereas contrasting positive vs. negative words showed subthreshold increased activation in the (para)hippocampal area. Cortisol secretion was significantly lower after TSD. Decreased voxel-by-voxel [ 11 C]raclopride binding potential (BP ND ) was observed in left caudate. TSD induces widespread cognitive, neurophysiologic and endocrine changes in healthy adults, characterized by reduced cognitive functioning, despite increased regional brain activity. The blunted HPA-axis response together with altered [ 11 C]raclopride binding in the basal ganglia indicate that sustained wakefulness requires involvement of additional adaptive biological systems.
Citation: Klumpers UMH, Veltman DJ, van Tol M-J, Kloet RW, Boellaard R, Lammertsma AA, et al. (2015) Neurophysiological Effects of Sleep Deprivation in Healthy Adults, a Pilot Study. PLoS ONE 10(1): e0116906. https://doi.org/10.1371/journal.pone.0116906
Academic Editor: Hengyi Rao, University of Pennsylvania, UNITED STATES
Received: August 17, 2013; Accepted: December 16, 2014; Published: January 21, 2015
Copyright: © 2015 Klumpers et al. This is an open access article distributed under the terms of the Creative Commons Attribution License , which permits unrestricted use, distribution, and reproduction in any medium, provided the original author and source are credited
Funding: This study was supported in part by ZONMW (Dutch Organization for Health Research and Development), The Netherlands, grant no. 016.066.309, to Dr. Ronald Boellaard. The funders had no role in study design, data collection and analysis, decision to publish, or preparation of the manuscript.
Competing interests: The authors have declared that no competing interests exist.
Introduction
Lack of sleep is a common condition in everyday life, either related to psychosocial demands or related to working shift hours. In healthy individuals, this may induce decreased alertness and vigilance, together with a general decline in mood. Total sleep deprivation (TSD) has been associated with general psychomotor slowing and diminished cognitive performance [ 1 , 2 ]. In affective disorders, only one night of sleep deprivation may improve mood in 40–60% of subjects with major depressive disorder [ 3 ], whereas bipolar patients may even turn into (hypo)mania [ 4 ]. Thus, in humans, sleep deprivation is clearly related to altered emotional and affective functioning.
From an evolutionary perspective, staying awake has served to guard against outside threats, requiring increased alertness. Motivational control over the waking state is necessary and presumed to be modulated by top-down cortical control systems, involving prefrontal executive regions [ 5 ]. Using [ 18 F]-2-fluoro-2-deoxy-D-glucose ([ 18 F]FDG) as a ligand in positron emission tomography (PET) studies, sleep deprivation has been associated with reduced metabolic activity in a network of brain regions, including prefrontal and limbic regions, the thalamo-basal ganglia circuit, and cerebellum [ 6 , 7 ]. Neurophysiologically, dopamine (DA) release is supposed to increase wakefulness, partly through the D2 receptor [ 8 , 9 , 10 ] and partly by acting as a stimulator of corticotropin releasing hormone (CRH) [ 11 ]. Ultimately, CRH releases cortisol from the adrenal cortex via the hypothalamic pituitary adrenal (HPA) axis, a key endocrine response mechanism to a stressful situation. These effects are superimposed upon the circadian rhythm of the HPA axis, and largely controlled by the central body clock, the suprachiasmatic nucleus (SCN). HPA axis functioning can be assessed by the cortisol awakening response (CAR), reflecting the natural HPA response to stress of sleep-wake transitions [ 12 ]. It is unknown, however, how cortical, dopaminergic and HPA axis activities interact to maintain wakefulness. Studying their interaction may also provide insight into the pathophysiology of depressive disorder, with its frequently occurring sleeping problems and HPA-axis hyperactivity [ 13 , 14 ].
The purpose of this pilot study was to assess how the healthy brain responds to TSD and how compensatory and regulatory stress mechanisms may interact as opposed to future clinical studies in mood disorder. It was hypothesized that wakefulness would be associated with an increase in dopamine release and CRH activation, in the presence of altered emotional functioning.
Materials and Methods
Participants.
Twelve healthy adults (6 female, mean age 29.2 ± 10.2 years; 6 male, mean age 28.5 ± 4.8 years) were recruited through newspaper advertisements. Exclusion criteria included a lifetime history of psychiatric disorders, as assessed by Mini international neuropsychiatric interview [ 15 ] and reported contacts with mental health counselors, previous use of psychotropic medication known to interfere with the dopaminergic system, 1 st degree relatives with psychiatric disorder, somatic disorders, pregnancy, use of sleep medication and past or current abuse of psychoactive drugs. All subjects were good sleepers, defined as feeling rested after a night’s sleep, and in good physical health as assessed by medical history, physical examination and routine laboratory tests. On the night preceding TSD, subjects slept 6.6 ± 1.1 hours. Mean body mass index was 21.0 ± 1.4 kg·m −2 , 2 were cigarette smokers (10 per day), and 10 consumed alcohol (1.5 ± 1.1 units day).
Ethics Statement
Written informed consent was obtained from all participants. The study protocol was approved by the Medical Ethics Review Committee of the VU University Medical Center in Amsterdam.
Design and Procedure
Cortisol saliva was collected on both days. At baseline (day 1), after a regular night of sleep at home, all subjects underwent functional magnetic resonance imaging (fMRI) scanning in the morning, followed by a 60min [ 11 C]raclopride PET scan. After this scanning session, participants returned to their daily activities, including study and/or work. They returned to the hospital at 22.00h for effectuation of total sleep deprivation. During the night, subjects were monitored by a trained observer and engaged in reading, conversation, short walks on the ward, and board games in a well-lit room. At arrival, urine toxicology was screened and found negative for a subset of dopaminergic and wake enhancing drugs, including cocaine, tetrahydrocannabinol (THC) and amphetamines. Use of alcohol, caffeinated beverages and smoking was prohibited during the night, as on both days in-between scan experiments. At day 2, a light meal was served at 6.00h. After having been awake for about 25 hours, fMRI scanning was repeated, followed by a second [ 11 C]raclopride PET scan for all participants. After finishing the scan sessions, subjects were asked to stay awake during the remainder of the day, and to postpone sleep until the evening.
Psychometric Data
Depressive symptoms over the prior week were assessed using the Beck Depression Inventory [ 16 ]. At baseline and before scanning, trait and state anxiety were measured using the Spielberger State-Trait Anxiety Inventory (STAI) [ 17 ]. During sleep deprivation, self and observer based visual analogue scales (VAS) were registered every 3 hours, starting at 24.00h and finishing at 12.00h, documenting mood, interest, motor inhibition, speed of thought, self appreciation, energy level and concentration on a scale from 0–100. Psychometric data were analyzed using Statistical Package for the Social Sciences (SPSS) version 15.0 for Windows (SPPS Inc, Chicago, Illinois, USA), using Repeated Measures ANOVA.
Cortisol Measurements
Data acquisition..
At the baseline interview, participants were instructed to collect saliva samples using Salivettes (Starstedt, Germany)[ 18 , 19 ], at 7 time points per day. One hour cortisol awakening response (CAR) measurements included three sampling points, immediately after awakening (T1), at +30min (T2) and at +60min (T3). Additional saliva samples were taken at +90min (T4) after awakening, at 14.00h (T5), 17.00h (T6) and 23.00h (T7). Subjects were instructed to write down the exact sampling time. On the following day, samples were collected at identical time points (T8–T14). Eating, smoking, drinking tea or coffee or brushing their teeth was prohibited within 15min before sampling. No dental work was allowed within 24 hours prior to sampling. Samples were stored in a refrigerator and returned by the participant or by regular mail. Salivettes were centrifuged at 2000g for 10min, aliquoted and stored at −80°C. Free cortisol analysis was performed by competitive electrochemiluminescence immunoassay (Architect, Abbott Laboratories, Illinois, USA) [ 20 ]. The lower limit of quantification was 2.0nmol·L −1 , the intra- and inter-assay variability coefficients were less than 9 and 11%.
Data analysis.
The CAR area under the curve (AUC), with respect to increase (AUC I ) and to ground (AUC G ), was calculated. AUC I is calculated with reference to the baseline measurement at T1, ignoring the distance from zero for all measurements, and emphasizing change over time. AUC G is the total area under the curve of all measurements [ 21 ]. The mean increase in the 1 st hour (MnInc) was calculated by subtracting the baseline value at T1 from the mean of the subsequent values at T2 and T3. Using the real sampling time at T2, T3, T9 and T10, cortisol levels were interpolated using piecewise linear spline to +30 and +60min, in order to derive the individual CAR AUC for identical time points on both days [ 22 ]. For AUC G T1-T7 and T8-T14, mixed model analysis was used to include time points available, with missing values being interpolated [ 23 ].
Task design.
We used a semantic emotional classification task adapted from Murphy [ 24 ] and Elliot [ 25 ], where subjects had to respond as quickly as possible to affective target stimuli and ignore distracter stimuli. The fMRI study consisted of two task sessions (runs), one to be executed at baseline and one after sleep deprivation. Each participant therefore performed two versions of the task, their order randomized across subjects. Each task comprised a blocked design with 16 blocks, programmed in E-prime software (Psychology Software Tools, Inc., Pittsburgh, PA, USA). The first two blocks were practice blocks while being in the magnet, to become acquainted with the task and to reduce anticipation anxiety. Within each session, eight different task conditions were presented twice in a pseudo-randomized order, to generate 16 blocks ( Table 1 ). In each block, 22 trials were presented in a randomized order, half of these being targets, and the other half consisting of distracters. Targets and distracters were defined on the basis of emotional valence, with happy (positive (P)), sad (negative (N)), or neutral (O) words as targets, presented with one of the other categories as distracters (e.g. positive targets with negative distracters). All the words were selected from the Centre for Lexical Information (Celex) Database [ 26 ], and matched for frequency of written use and word length. Affective words were selected on high emotional impact (positive words 6.0 ± 1.6 letters, intensity 2.2 ± 0.5; negative words 5.7 ± 0.4 letters, intensity 5.9 ± 1). A baseline neutral condition was included, where targets and distracters were defined on the basis of physical properties (italic (I) vs. regular (R) font), providing similar visual input. Each of the 16 blocks started with a written instruction for a fixed 5s, followed by a 1s rest, in which subjects were instructed to respond as fast as possible to the appropriate task condition by pressing a button with the preferred index finger. Following a fixation cross for 800ms, a word was shown for 500ms to which subjects were allowed to respond within an additional fixed inter-stimulus interval of 900ms. After pressing, the word was no longer visible. At the end of a block, a 1s rest was included prior to the next block.
- PPT PowerPoint slide
- PNG larger image
- TIFF original image
https://doi.org/10.1371/journal.pone.0116906.t001
T1-weighted MRI scans were acquired using a 1.5T Sonata MR system (Siemens Medical Solutions, Erlangen, Germany) to exclude anatomical abnormalities and for PET and fMRI co-registration purposes. A sagittal 3D gradient-echo T1-weighted image was acquired using the following sequence: repetition time (TR) = 2.7ms, echo time (TE) = 3.97ms, matrix 256×160, voxel size 1×1×1.5mm 3 . Echo-planar images (EPI) were obtained using a T2*-weighted gradient echo sequence TR = 2.18s, TE = 45ms, 35 axial slices; voxel size 3×3×3mm 3 , flip angle 90°, matrix 64×64). For the fMRI task, stimuli were projected onto a screen at the end of the scanner table, visible through a mirror mounted above the subject’s head. Two magnetic field compatible response boxes were used to record the subject’s responses.
Data processing.
Functional imaging data were preprocessed and analyzed using Statistical Parametric Mapping (SPM) software (SPM8, Wellcome Trust Neuroimaging Centre, London, UK), implemented in Matlab 7.1.0 (The MathWorks Inc., Natick, MA, USA). Preprocessing included reorientation of the functional images to the anterior commissure, slice time correction, image realignment, co-registration of the T1 scan to the mean image, warping of the co-registered T1 image to Montreal Neurological Institute (MNI) space as defined by SPM’s T1 template, applying the transformations to the slice-timed and realigned images, reslicing to voxels of 3×3×3mm 3 and applying spatial smoothing using an 8mm full width at half maximum (FWHM) Gaussian kernel. Subject movements of more than 3mm in more than one direction resulted in exclusion of data.
In the first level analysis, scanner drifts were modeled using a high pass filter with a cut off of 128s. For each regressor, the onset of the block and the duration of the total block were modeled as a block design, consisting of 22 trial words × [800msec (fixation cross) + 500msec (word presentation) + 900msec (maximum time to press the button)] per word, plus 21 intervals × 32 msec (refresh rate word in scanner), totaling 49.072 ms. Task instructions were modeled separately as a regressor of no interest ( Table 1 ).
The following contrast images were computed:
- 1). [−2 1 0 1 0 0 0 0] positive classification vs. baseline, in which the positive-neutral (P-O) and neutral-positive (O-P) word pairs were grouped and contrasted to the baseline (italic-regular font pairs and vice versa).
- 2). [−2 0 1 0 1 0 0 0] negative classification vs. baseline, in which the negative-neutral (N-O) and neutral-negative (O-N) word pairs were grouped and contrasted to the baseline (italic-regular font pairs and vice versa).
- 3). [−2 0 0 0 0 1 1 0] both emotional valences vs. baseline, in which exclusively emotional valence pairs (P-N and N-P) were grouped and contrasted to the baseline (italic-regular font pairs and vice versa).
- 4). [−6 1 1 1 1 1 1 0] any emotional valence vs. baseline, in which all emotional valences (P-O, N-O, O-P, O-N, P-N and N-P) were grouped and contrasted to the baseline (italic-regular font pairs and vice versa).
These contrasts were defined for both pre-deprivation and post-deprivation sessions.
Next, on a second level, the contrast images for positive vs. baseline for the pre-deprivation session and the post-deprivation session were entered in a two-sample t -test, with session as dependent variable. Additionally, separate models were set up for negative vs. baseline, exclusively emotional valence pairs and any emotional valence vs. baseline. Due to the relative low number of subjects, no additional covariates were entered to these models.
The main effect of time (day 1 vs. day 2) was explored at a threshold of p uncorrected <0.005, with an extent threshold of 10 contiguous voxels. Additionally, correction for multiple comparisons was performed by applying Small Volume Correction (SVC) for regions of interest (ROIs) with known involvement in depression, sleep abnormalities and emotional attention. As described in the introduction, the following regions were selected: dorsolateral prefrontal cortex, subgenual cingulate, hippocampal gyrus/ amgydala and insula, defined using the Automated Anatomical Labeling (AAL) system as implemented in the WFU-pickatlas toolbox [ 27 ]. Effects occurring in these regions were thus followed up using SVC-correction and results are reported at a Family Wise error (FWE) corrected p-value <.05. Psychometric and performance data (correct responses, false alarms, misses and mean response time for events (RT)) for both days were likewise analysed using paired sample t -testing.
[ 11 C]Raclopride PET
[ 11 C]Raclopride scans were performed on an ECAT EXACT HR+ scanner (Siemens/CTI, Knoxville, TN, USA). Participants were studied at rest, in supine position, with a nurse nearby and ice cubes in both hands to prevent them from falling asleep. Head movement was restricted by a head immobilization device and Velcro tape. A venous catheter was placed in the forearm for [ 11 C]raclopride infusion. A 10min 2D transmission scan using three rotating 68 Ge/ 68 Ga sources was acquired for photon attenuation correction. 370MBq [ 11 C]raclopride was dissolved in 5mL saline and administered by an infusion pump (Med-Rad, Beek, The Netherlands), at a rate of 0.8mL·s −1 , followed by a 35mL saline flush at a rate of 2.0mL·s −1 . Meanwhile, a 60min dynamic 3D raclopride scan was acquired, consisting of 20 frames with progressively increasing frame lengths (1×15, 3×5, 3×10, 2×30, 3×60, 2×150, 2×300, 4×600s). All PET sinograms were normalized and corrections were applied for decay, dead time, attenuation scatter and randoms. Emission data were reconstructed using FORE+2D filtered back projection [ 28 , 29 ] applying a 5.0mm Hanning filter with a Y-offset of 4cm and a 2.123 zoom. Frames 12–20 were summed (i.e. 5–60min after injection) to create a single frame emission sinogram with high count statistics. Reconstruction of this emission sinogram was performed using ordered-subset expectation maximization (OSEM) with 4 iterations and 16 subsets. OSEM images underwent a 5mm FWHM Gaussian post smoothing, to obtain a transaxial spatial resolution of 7mm FWHM, equal to that of filtered back projected (FBP) images. Final images consisted of 63 planes of 128×128 voxels, each 2.4×2.4×2.4 mm 3 .
All structural MRI scans were rotated to the axial (horizontal) plane, parallel to the anterior and posterior commissure (AC–PC) line. To correct for possible motion, each frame (1–20) was coregistered to the summed image over frames 12–20. These motion corrected PET images were subsequently coregistered to the realigned MRI scan using Volume Imaging in Neurological Research (VINCI) software [ 30 ].
Kinetic analysis.
Mean non-displaceable binding potential (BP ND ) was used as a measure of dopamine D2/D3 receptor availability. Using the in-house developed software package PPET [ 31 ], parametric BP ND images were generated using receptor parametric imaging (RPM2), a basis function implementation of the simplified reference tissue model (SRTM) [ 32 ]. Cerebellum grey matter was used as reference tissue, for which automated cerebellar volumes of interest (VOIs) were defined using partial volume effect (PVE) lab [ 33 ]. This analysis also provided parametric R 1 images, representing local tracer delivery relative to that to the cerebellar reference region. Basis function settings used were: start exponential = 0.05min −1 , end = 0.5min −1 , number of basis functions 32.
Statistical parametric mapping.
Parametric BP ND images were analyzed using SPM8. After spatial preprocessing, including reorientation and normalization to MNI space, images were analyzed on a voxel by voxel basis, using a basal ganglia mask created with WFU Pickatlas software [ 27 ]. No proportional scaling was applied. SPM RPM2 and R 1 BP ND images were entered in paired sample t -tests. The threshold was set at p uncorrected ≤0.005 with an extent threshold of 10 voxels.
At baseline, depressive symptoms were low to absent (BDI score 1.8 ± 2.0). Using the Spielberger State-Trait Inventory (STAI), containing 20 items to be scored on a four-point Likert scale (range 20–80), mean trait anxiety score was 29.4 ± 4.8 and state anxiety at baseline scanning 30.4 ± 3.9. During the TSD night, VAS energy levels declined significantly (F(1,11) 20.2, p = 0.001), in line with decreased concentration (F(1,11) 10.6, p = 0.01), speed of thought (F(1,11) 12.0, p = 0.007), and increased perceived motor retardation (F(1,11) 12.0, p = 0.007), but not significantly for mood (F(1,11) 2.9, p = 0.122). STAI scores indicated a trendwise increased anxiousness after TSD, 36.3 ± 10.7 ( p = 0.068).
Cortisol Data
After TSD, CAR AUC I and AUC G showed significant blunting ( p = 0.029 and p = 0.022, respectively) ( Table 2 , Fig. 1 ). On day 1, nine subjects showed a rise in cortisol during the first hour after awakening, compared with a much smaller increase in five subjects after TSD, signified by a decreasing MnInc CAR. Similarly, cortisol AUC G T1-7 vs. T8-14 showed a robust decline after TSD. Cortisol levels were normally distributed on both days and showed no significant gender differences. Evening cortisol was not discriminating.
Individual saliva cortisol curves (grey line) and cortisol mean value (nmol/L) per Tx sampling point (solid line). Day 1 shows baseline cortisol sampling at T1-T7, day 2 shows effects of one night of total sleep deprivation on cortisol levels at T8-T14. T1, 2 and 3 comprise the cortisol awakening response (CAR). T8, 9 and 10 are sampled at identical time points the following day. T5 and T12 are sampled at 14.00hr, T6 and T13 at 17.00hr and T7 and T14 at 23.00hr. p values show effects of TSD, # p = 0.016.
https://doi.org/10.1371/journal.pone.0116906.g001
https://doi.org/10.1371/journal.pone.0116906.t002
Twelve data sets were available on day 1, and 11 on day 2 due to scanner logistic problems. After TSD, subjects were significantly slower in reacting during the neutral condition ( p = 0.043), but also to positive targets with a neutral distracter ( p = 0.008). The proportion of correct versus false answers decreased trendwise for neutral words ( p = 0.082) and for positive targets with a negative distracter ( p = 0.079) ( Table 3 ). Post hoc , results were additionally analyzed using general linear model statistics (GLM). When performing multivariate testing, the effect of sleep deprivation on reaction time for emotional words was significant at F(1,20) = 34.14, p <0.001; the effect of time for sleep deprivation was significant at F(1,20) = 5.78, p = 0.037, indicating that participants were slower at day 2, due to sleep deprivation. The interaction effect of sleep deprivation on emotion* time was not significant (F(1,20) = 0.81, p = 0.475), indicating that the general slowing following deprivation was common for all emotions presented.
https://doi.org/10.1371/journal.pone.0116906.t003
After 25 hours of wakefulness, the neutral condition showed no significant activation differences at a group level ( Table 4 ). Evaluation and processing of positive words was associated with increased bilateral prefrontal activation in addition to increased activation of left medial prefrontal working memory areas ( Fig. 2A ). Left DLPFC activation remained significant after Small Volume Correction (SVC; AAL p FWE 0.02). Processing of negative words was associated with increased activity in left insular area, but this effect did not survive SVC. During conditions containing emotional words only, viz. positive targets and negative distracters (P-N), or vice versa (N-P), left insular, limbic and parahippocampal lobes were activated, as well as right parietal lobe ( Fig. 2B ), showing SVC subthreshold increased activation in the hippocampal/parahippocampal region. All emotional conditions (i.e. target and/or distracter) resulted in increased activation in the anterior part of the left insula (AAL p FWE 0.043), mainly driven by the response to words with a negative valence, in addition to activation of the parietal lobe.
p <0.005, extent threshold 10 voxels. A and B are task related fMRI results, showing increased prefrontal and limbic activation respectively, in the conditions (A) positive valence versus baseline and (B) both emotional valences. C is a [ 11 C]raclopride PET image, showing decreased voxel-by-voxel RPM2 binding potential (BP ND ) in nucleus caudatus in n = 8. At the bottom right is the Z-score scale depicted.
https://doi.org/10.1371/journal.pone.0116906.g002
https://doi.org/10.1371/journal.pone.0116906.t004
[ 11 C]Raclopride
A subset of 8 paired data sets was available due to a failed synthesis (1 TSD scan) and technical problems with 1 baseline and 2 TSD scans. For n = 8, injected masses of raclopride were 2.36 ± 1.08 and 1.45 ± 0.55μg, on days 1 and 2 respectively ( p = 0.06) and injected doses of [ 11 C]raclopride were 378 ± 12 and 390 ± 19MBq on days 1 and 2, respectively ( p = 0.230). TSD resulted in a significantly decreased voxel-by-voxel based BP ND in left caudate nucleus, as shown in Table 4 and Fig. 2C . In addition, there was a TSD induced decrease in R 1 in right caudate nucleus.
Clinical Interactions
Post hoc we tested for correlations for TSD related changes in cortisol AUC, regions of interest (ROI) based BOLD response to emotional words, and altered [ 11 C]raclopride binding, using anatomical automatic labeling (AAL) defined striatal regions, according to WFU Pick atlas [ 27 ]. No statistically significant correlations were observed.
In the present study the effects of total sleep deprivation on stress regulating brain systems in healthy subjects were investigated as preliminary work for a TSD study in mood disorder. During a sleep deprived night, VAS scores on energy, concentration and speed of thought, but not mood, declined significantly. Although at baseline participants were not clinically depressed or overly anxious, as witnessed by BDI and STAI-scores, validated instruments like the Positive and Negative Affect Schedule (PANAS)[ 34 , 35 ] and the Profile of Mood State (POMS)[ 36 ] could have been used to score a broad range of mood states, both at baseline and during the night of sleep deprivation and the subsequent day.
After 24 hours of prolonged wakefulness, significant blunting of the cortisol awakening response (CAR) and secretion over the day (AUC G ) were found. Normally, under the influence of the SCN, HPA activity increases during the night, resulting in a cortisol rise two to three hours after sleep onset, which continues to rise into the early waking hours [ 12 , 14 ]. The present results indicate robust attenuation of the HPA-mediated stress response after TSD, congruous with decreasing VAS scores and lowered arousal, which may be due to the absence of the initial physiological awakening response [ 12 , 37 ]. These findings are in line with Vzontgas and colleagues, finding lowered, albeit not significantly, 24 hour plasma cortisol levels in blood in a laboratory setting in a group of 10 men [ 38 ].
In the present study, no significantly altered cortisol levels were found after 14.00h (T5-T12). Evening cortisol, indicating return to baseline levels, was slightly lower than those reported by Vreeburg [ 19 ] in healthy subjects.
In order to investigate effects of TSD on processing of both positive and negative stimuli, as well as on cognitive inhibition, we chose to adapt the Murphy and Elliott fMRI paradigm [ 24 , 25 , 39 , 40 ], as this task was originally developed to investigate emotional bias in mood disorders in the context of cognitive processing. After TSD, in healthy adults, task performance during fMRI was slower, indicating that TSD overruled any learning or practice effects. Slowing of task performance after TSD is in line with previous reports and likely due to loss of sustained attention and vigilance [ 41 ]. Slowing was particularly evident for positive targets with a neutral distracter. Accuracy was trendwise decreased for the neutral (italic vs. regular font) condition and for positive targets with negative distracters, suggesting decreased sensitivity to detect positive valence. On processing emotionally salient versus neutral words, TSD was associated with increased left dorsolateral prefrontal activity, suggesting increased mental effort to perform semantic judgements and to maintain control, in a setting of less efficient functional circuitry. Although we do not intend to overstate the relevance of these findings in this emotionally healthy group, cognitive biases in depressive disorder are thought to reflect maladaptive bottom-up processes, which are generally perpetuated by weakened cognitive control [ 42 ]. Processing of solely affective stimuli (target and distracter) showed subthreshold increased activation in the left parahippocampal /hippocampal region. Activation of the subgenual gyrus and amygdala was remarkably absent, though for amygdala this is line with findings by Elliott et al., [ 25 ], fostering [presumably reflecting] a lower affective salience for words compared to pictures. Processing of any affective stimulus (target and/or distracter) showed increased activation of the anterior part of the insula in a context of performance anxiety as indicated by trendwise increased STAI scores in these healthy, but weary adults [ 43 ]. Activation of the insula was mainly driven by the response to words with a negative valence, suggestive of an increased effort to handle negative affect [ 1 ] and in line with the insular function of emotional interference resolution in working memory [ 44 ]. With due caution, we propose that these neural responses reflect modulation of cognitive performance by emotional tone. Therefore, these regions likely represent an interface between cognition and emotion processing [ 25 ].
After TSD, voxel-by-voxel based BP ND of [ 11 C]raclopride was significantly decreased in left caudate, which is partly in accordance with a report by Volkow [ 9 ]. This was not explained by regional altered delivery (R 1 ) of the tracer, although metabolic activity in the cerebellar reference tissue may be altered after sleep deprivation [ 6 , 7 ]. A reduction in [ 11 C]raclopride specific binding is consistent with either an increase in dopamine release, or a decreased affinity of the synaptic D2/D3 receptor in these regions [ 45 ], which may be due to internalization of receptors [ 46 ]. This could not be determined on the basis of our design, and may have resulted from a combination of these factors.
Using both [ 11 C]raclopride and a dopamine transporter blocking radioligand, [ 11 C]methylphenidate, Volkow [ 10 ] argued TSD induced decreased [ 11 C]raclopride binding not to be due to increased dopamine availability, but to decreased affinity of the D2/D3 receptor, resulting in dopamine receptor downregulation in the synaptic cleft. As dopamine D2 receptors are thought to be involved in wakefulness, and partially responsible for maintaining arousal and alertness [ 8 , 47 ], the present reduced VAS on energy and concentration and efficiency in fMRI task performance, are in line with D2 down-regulation. This would further be exemplified by the blunted cortisol response, since dopaminergic stimulation of the HPA axis is mediated through D1 and D2 receptors [ 11 ]. Decreased affinity in the head of the left caudate could be in line with increased difficulty in controlling word interference from task unrelated processing [ 48 ], explaining both the general slowing and increased prefrontal activity. However, we were not able to corroborate this explanation in a correlational analysis, which may be primarily due to insufficient power, but may also indicate that regional brain activation as measured with fMRI is not tightly coupled to either striatal dopaminergic transmission or HPA axis activity. Excluding two smokers did not change results significantly, although smoking may influence dopamine release and therefore raclopride binding [ 49 ].
Clinical Relevance
Individual vulnerability to sleep deprivation is known to be variable [ 3 ]. From the present study, it cannot be ruled out that decreased D2 receptor affinity is the brain’s response to initially increased dopamine levels, induced by TSD. Blunting of the HPA axis response may reflect the absence of awakening stress and possibly explain some of the beneficial effects of sleep deprivation in depressive mood disorder.
Limitations
This pilot study in healthy adults contains several potential limitations. In view of our modest sample size and fixed-order design, the current results are in clear need of replication.
Regarding baseline characteristics, the participants’ number of hours of sleep was adequate at the start of the experiment, but we did not control objectively for sleep quality and duration. Baseline CAR may have been affected by waking up earlier, or by the excitement of taking part in a research study, which may have released additional ACTH [ 50 ]. A higher CAR has been associated with shorter sleep duration [ 51 ]. However, excluding three subjects who slept 6 hours or less, did not have a major effect on the CAR ( p = 0.022). During the night, participants were kept in a well-lit room. Melatonin suppression may have dampened the SCN-mediated CRH response.
For our fMRI runs, we have chosen to adapt the original Murphy and Elliott task [ 24 , 25 ], who described their paradigm to investigate emotional bias in depressive disorder as a go/no-go task. However, go/no-go paradigms do not typically feature an even split of valid and invalid targets, and therefore we have renamed the task as a semantic affective classification task. The task was modeled as a block design, and because the inter-stimulus interval (ISI) was fixed, could not be analyzed as an event-related design. Evidently, a block design is preferable when sample sizes are modest, as it is generally more robust [ 52 ], although it lacks the flexibility of event-related designs. Therefore, for assessing individual cognitive and emotional responses in e.g. a patient population, an event-related design would be more appropriate [ 53 ]. Finally, for our voxel-based analyses we set an a priori threshold of p = 0.005 and 10 voxels to obtain a reasonable balance between Type I and Type II error [ 54 ], again highlighting the need for a replication in a larger sample.
With respect to mood enhancers, other drugs of abuse were not tested for. At baseline, we did not control for caffeine use at home before the start of the experiments. Caffeine evokes its stimulating effects through blockade of the adenosine receptor [ 55 ], which in turn is involved in the control of dopamine release [ 56 ]. As raclopride is a dopamine receptor antagonist, in theory, TSD induced changes in raclopride binding may therefore have been underestimated.
Although changes in [ 11 C]raclopride BP ND clearly show a dose dependent relationship with extracellular DA levels, the nature of this relationship is complex [ 57 ]. [ 11 C]Raclopride BP ND does not differentiate between binding to receptors in high or low affinity states, whereas endogenous dopamine is mainly conveyed by high affinity state receptors [ 58 ], acting on pre- and postsynaptic (extra)-striatal dopaminergic D1 receptors to bring about its effect [ 59 ]. Therefore, dopaminergic effects due to TSD may have been underestimated and future research should resolve this issue, for example by comparing [ 11 C]raclopride to the purported high-affinity ligand [ 11 C]PHNO [ 60 ]. As [ 11 C]raclopride scans were performed in the second half of the morning, and the time sequence of dopamine release is not known, effects may have been either over- or underestimated. A variable response to TSD is in line with observations in depressed patients, where the therapeutic response to TSD may vanish within hours to a day [ 3 ].
Sleep deprivation in healthy adults induces widespread neurophysiological and endocrine changes, characterized by impaired cognitive functioning, despite increased regional brain activity. Our pilot findings indicate that activation of the dopaminergic system occurs together with a blunted cortisol response, suggesting augmented motivational top down control and requiring increased involvement of prefrontal and limbic cortical areas. Sustained wakefulness requires the involvement of compensatory brain systems, and may help to understand the therapeutic effects of sleep deprivation in affective disorders.
Acknowledgments
The authors thank Ms Marieke Mink for accompanying the participants to the PET scanning sessions, Dr. Marjan Nielen for help in designing the fMRI task, Dr. Sophie Vreeburg for help in interpreting cortisol data, Dr. Adriaan Hoogendoorn for statistical support, Neuroradiology staff for interpretation of MRI scans, staff of the department of Nuclear Medicine & PET Research for tracer production, technical assistance and data acquisition and staff of the VU Medical Center Neuroendocrine lab for cortisol saliva analysis.
Author Contributions
Conceived and designed the experiments: UK DV MJT RK RB AAL WH. Performed the experiments: UK DV MJT RK. Analyzed the data: UK DV MJT RK RB AAL WH. Contributed reagents/materials/analysis tools: UK DV MJT RK RB AAL WH. Wrote the paper: UK DV MJT RK RB AAL WH.
- View Article
- PubMed/NCBI
- Google Scholar
- 36. McNair DM, Lorr M, Droppleman LF (1971) Manual for the Profile of Mood States.
- 53. Huettel SA, Song AW, McCarthy G (2009) Functional Magnetic Resonance Imaging. Sunderland MA: Sinauer Associates.
- Search Menu
- Advance Articles
- Supplements
- Editor's Choice
- Virtual Issues
- Virtual Roundtables
- Abstract Supplements
- Basic Science
- Circadian Disorders
- Cognitive, Affective and Behavioral Neuroscience of Sleep
- Neurological Disorders
- Sleep Across the Lifespan
- Sleep and Metabolism
- Sleep Disordered Breathing
- Sleep Health and Safety
- Author Guidelines
- Instructions for Reviewers
- Submission Site
- Open Access Options
- Additional Resources
- Self-Archiving Policy
- About SLEEP
- Editorial Board
- Dispatch Dates
- Permissions
- Advertising & Corporate Services
- Journals Career Network
- Reprints and ePrints
- Sponsored Supplements
- Journals on Oxford Academic
- Books on Oxford Academic
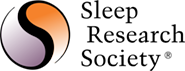
Article Contents
- < Previous
Effects of Sleep Deprivation on Performance: A Meta-Analysis
- Article contents
- Figures & tables
- Supplementary Data
June J. Pilcher, Allen I. Huffcutt, Effects of Sleep Deprivation on Performance: A Meta-Analysis, Sleep , Volume 19, Issue 4, June 1996, Pages 318–326, https://doi.org/10.1093/sleep/19.4.318
- Permissions Icon Permissions
To quantitatively describe the effects of sleep loss, we used meta-analysis, a technique relatively new to the sleep research field, to mathematically summarize data from 19 original research studies. Results of our analysis of 143 study coefficients and a total sample size of 1,932 suggest that overall sleep deprivation strongly impairs human functioning. Moreover, we found that mood is more affected by sleep deprivation than either cognitive or motor performance and that partial sleep deprivation has a more profound effect on functioning than either long-term or short-term sleep deprivation. In general, these results indicate that the effects of sleep deprivation may be underestimated in some narrative reviews, particularly those concerning the effects of partial sleep deprivation.
Email alerts
Citing articles via, looking for your next opportunity.
- Recommend to Your Librarian
- Advertising and Corporate Services
Affiliations
- Online ISSN 1550-9109
- Print ISSN 0161-8105
- Copyright © 2024 Sleep Research Society
- About Oxford Academic
- Publish journals with us
- University press partners
- What we publish
- New features
- Open access
- Institutional account management
- Rights and permissions
- Get help with access
- Accessibility
- Advertising
- Media enquiries
- Oxford University Press
- Oxford Languages
- University of Oxford
Oxford University Press is a department of the University of Oxford. It furthers the University's objective of excellence in research, scholarship, and education by publishing worldwide
- Copyright © 2024 Oxford University Press
- Cookie settings
- Cookie policy
- Privacy policy
- Legal notice
This Feature Is Available To Subscribers Only
Sign In or Create an Account
This PDF is available to Subscribers Only
For full access to this pdf, sign in to an existing account, or purchase an annual subscription.
Effects of Sleep Deprivation
- First Online: 22 February 2024
Cite this chapter
- Andrea Cecilia Toscanini 2 &
- Rosa Hasan 3
139 Accesses
This chapter aims to inform the professional about the effects of sleep deprivation on the individual’s health, quality of life, and performance. In addition, it discusses the possible associations of insomnia with clinical or psychiatric comorbidities. Bidirectional relationships between different comorbidities and sleep will also be the focus of this chapter. Since the patient with insomnia often also has another comorbidity, understanding these associations and relationships is of great value in conducting the clinical case.
This is a preview of subscription content, log in via an institution to check access.
Access this chapter
- Available as EPUB and PDF
- Read on any device
- Instant download
- Own it forever
- Durable hardcover edition
- Dispatched in 3 to 5 business days
- Free shipping worldwide - see info
Tax calculation will be finalised at checkout
Purchases are for personal use only
Institutional subscriptions
Sleep difficulties and sleep deprivation can lead to worsening of anxiety symptoms, including panic attacks, in patients with panic disorder.
Aldabal, L., & Bahammam, A. S. (2011). Metabolic, endocrine, and immune consequences of sleep deprivation. Open Respiratory Medicine Journal, 5 , 31–43.
Article PubMed PubMed Central Google Scholar
Baumann, C. R., Kilic, E., Petit, B., et al. (2006). Sleep EEG changes after middle cerebral artery infarcts in mice: Different effects of striatal and cortical lesions. Sleep, 29 (10), 1339–1344.
Article PubMed Google Scholar
Biegańska, K., Sokołowska, P., Jöhren, O., & Zawilska, J. B. (2012). Orexin A suppresses the growth of rat C6 glioma cells via a caspase-dependent mechanism. Journal of Molecular Neuroscience, 48 (3), 706–712.
Bishir, M., Bhat, A., Essa, M. M., Ekpo, O., Ihunwo, A. O., Veeraraghavan, V. P., Mohan, S. K., Mahalakshmi, A. M., Ray, B., Tuladhar, S., Chang, S., Chidambaram, S. B., Sakharkar, M. K., Guillemin, G. J., Qoronfleh, M. W., & Ojcius, D. M. (2020). Sleep deprivation and neurological disorders. BioMed Research International, 2020 , 5764017. https://doi.org/10.1155/2020/5764017 . PMID: 33381558; PMCID: PMC7755475.
Bixler, E. O., Vgontzas, A. N., Lin, H. M., Calhoun, S. L., Vela-Bueno, A., & Kales, A. (2005). Excessive daytime sleepiness in a general population sample: The role of sleep apnea, age, obesity, diabetes, and depression. The Journal of Clinical Endocrinology and Metabolism, 90 (8), 4510–4515.
Buckley, A. W., Rodriguez, A. J., Jennison, K., et al. (2010). Rapid eye movement sleep percentage in children with autism compared with children with developmental delay and typical development. Archives of Pediatrics & Adolescent Medicine, 164 (11), 1032–1037.
Article Google Scholar
Centers for Disease Control and Prevention (CDC). (2009). Perceived insufficient rest or sleep among adults - United States, 2008. MMWR. Morbidity and Mortality Weekly Report, 58 (42), 1175–1179.
Google Scholar
Chalah, M. A., & Ayache, S. S. (2018). Is there a link between inflammation and fatigue in multiple sclerosis? Journal of Inflammation Research, 11 , 253–264.
Chattu, V. K., Sakhamuri, S. M., Kumar, R., Spence, D. W., BaHammam, A. S., & Pandi-Perumal, S. R. (2018). Insufficient Sleep Syndrome: Is it time to classify it as a major noncommunicable disease? Sleep Science, 11 (2), 56–64. https://doi.org/10.5935/1984-0063.20180013 . PMID: 30083291; PMCID: PMC6056073.
Chennaoui, M., Sauvet, F., Drogou, C., Van-Beers, P., Langrume, C., Guillard, M., et al. (2011). Effect of one night of sleep loss on changes in tumor necrosis factor alpha (TNF-alpha) levels in healthy men. Cytokine, 56 (2), 318–324. https://doi.org/10.1016/j.cyto.2011.06.002
Chien, K. L., Chen, P. C., Hsu, H. C., Su, T. C., Sung, F. C., Chen, M. F., et al. (2010). Habitual sleep duration and insomnia and the risk of cardiovascular events and all-cause death: Report from a community-based cohort. Sleep, 33 (2), 177–184.
Cortese, S., Faraone, S. V., Konofal, E., & Lecendreux, M. (2009). Sleep in children with attention-deficit/hyperactivity disorder: Meta-analysis of subjective and objective studies. Journal of the American Academy of Child and Adolescent Psychiatry, 48 , 894–908.
PubMed Google Scholar
Cournot, M., Ruidavets, J. B., Marquie, J. C., Esquirol, Y., Baracat, B., & Ferrieres, J. (2004). Environmental factors associated ` with body mass index in a population of southern France. European Journal of Cardiovascular Prevention and Rehabilitation, 11 (4), 291–297.
Currie, L. J., Bennett, J. P., Harrison, M. B., Trugman, J. M., & Wooten, G. F. (1997). Clinical correlates of sleep benefit in Parkinson’s disease. Neurology, 48 (4), 1115–1117.
Dong, H., Wang, J., Yang, Y. F., Shen, Y., Qu, W. M., & Huang, Z. L. (2019). Dorsal striatum dopamine levels fluctuate across the sleep–wake cycle and respond to salient stimuli in mice. Frontiers in Neuroscience, 13, 242 .
Duan, D., Kim, L. J., Jun, J. C., & Polotsky, V. Y. (2023). Connecting insufficient sleep and insomnia with metabolic dysfunction. Annals of the New York Academy of Sciences, 1519 (1), 94–117. https://doi.org/10.1111/nyas.14926 . Epub 2022 Nov 13. PMID: 36373239; PMCID: PMC9839511.
Essa, M. M., Moghadas, M., Ba-Omar, T., et al. (2019). Protective effects of antioxidants in Huntington’s disease: An extensive review. Neurotoxicity Research, 35 (3), 739–774.
Ferrie, J. E., Kivimaki, M., Akbaraly, T. N., Singh-Manoux, A., Miller, M. A., Gimeno, D., et al. (2013). Associations between change in sleep duration and infl ammation: Fi ndings on C-reactive protein and interleukin 6 in the Whitehall II study. American Journal of Epidemiology, 178 (6), 956–961. https://doi.org/10.1093/aje/kwt072
Ford, E. S., Cunningham, T. J., Giles, W. H., & Croft, J. B. (2015). Trends in insomnia and excessive daytime sleepiness among U.S. adults from 2002 to 2012. Sleep Medicine, 16 (3), 372–378.
Frey, D. J., Fleshner, M., & Wright, K. P. (2007). The effect of 40 hours of total sleep deprivation on infl ammatory markers in healthy young adults. Brain, Behavior, and Immunity, 21 (8), 1050–1057. https://doi.org/10.1016/j.bbi.2007.04.003
Gao, B., Cam, E., Jaeger, H., Zunzunegui, C., Sarnthein, J., & Bassetti, C. L. (2010). Sleep disruption aggravates focal cerebral ischemia in the rat. Sleep, 33 (7), 879–887.
Goldberg, G. R., Prentice, A. M., Davies, H. L., & Murgatroyd, P. R. (1988). Overnight and basal metabolic rates in men and women. European Journal of Clinical Nutrition, 42 (2), 137–144.
Grandner, M. A., Buxton, O. M., Jackson, N., Sands-Lincoln, M., Pandey, A., & Jean-Louis, G. (2013). Extreme sleep durations and increased C-reactive protein: Effects of sex and ethnoracial group. Sleep, 36 (5), 769–779. https://doi.org/10.5665/sleep.2646
Hafner, M., Stepanek, M., Taylor, J., Troxel, W. M., & van Stolk, C. (2017). Why sleep matters: The economic costs of insufficient sleep: A cross-country comparative analysis. Rand Health Quarterly, 6 (4), 11.
PubMed PubMed Central Google Scholar
Hagewoud, R., Havekes, R., Novati, A., Keijser, J. N., Van der Zee, E. A., & Meerlo, P. (2010). Sleep deprivation impairs spatial working memory and reduces hippocampal AMPA receptor phosphorylation. Journal of Sleep Research, 19 (2), 280–288.
Hakki Onen, S., Alloui, A., Jourdan, D., Eschalier, A., & Dubray, C. (2001). Effects of rapid eye movement (REM) sleep deprivation on pain sensitivity in the rat. Brain Research, 900 (2), 261–267.
Hampton, S. M., Morgan, L. M., Lawrence, N., et al. (1996). Postprandial hormone and metabolic responses in simulated shift work. Journal of Endocrinology, 151 (2), 259–267.
Hardeland, R., & Pandi-Perumal, S. R. (2005). Melatonin, a potent agent in antioxidative defense: Actions as a natural food constituent, gastrointestinal factor, drug and prodrug. Nutrition & Metabolism, 2 (1), 22.
Harvey, A. G., Schmidt, D. A., Scarna, A., et al. (2005). Sleep-related functioning in euthymic patients with bipolar disorder, patients with insomnia, and subjects without sleep problems. The American Journal of Psychiatry, 162 , 50–57.
Hayley, A. C., Williams, L. J., Kennedy, G. A., Berk, M., Brennan, S. L., & Pasco, J. A. (2014). Prevalence of excessive daytime sleepiness in a sample of the Australian adult population. Sleep Medicine, 15 (3), 348–354.
Herzog-Krzywoszanska, R., & Krzywoszanski, L. (2019). Sleep disorders in Huntington’s disease. Frontiers in Psychiatry, 10 , 221.
Jung, B., & Ahmad, N. (2006). Melatonin in cancer management: Progress and promise: Figure 1. Cancer Research, 66 (20), 9789–9793.
Kaminska, M., Kimoff, R. J., Schwartzman, K., & Trojan, D. A. (2011). Sleep disorders and fatigue in multiple sclerosis: Evidence for association and interaction. Journal of the Neurological Sciences, 302 (1–2), 7–13.
Kessler, R. C., Berglund, P., Demler, O., et al. (2005). Lifetime prevalence and age-of-onset distributions of DSM-IV disorders in the National Comorbidity Survey Replication. Archives of General Psychiatry, 62 (6), 593–602.
Kinucan, J. A., Rubín, D. T., & Ali, T. (2013). Sleep and inflammatory bowel disease: Exploring the relationship between sleep disturbances and infl ammation. Gastroenterology and Hepatology (New York), 9 (11), 718–727.
Kochanek, K. D., Murphy, S. L., Xu, J., & Arias, E. (2014). Mortality in the United States, 2013. NCHS Data Brief , (178), 1–8.
Kohyama, J., Anzai, Y., Ono, M., Kishino, A., Tamanuki, K., Takada, K., Inoue, K., Horiuchi, M., & Hatai, Y. (2018). Insufficient sleep syndrome: An unrecognized but important clinical entity. Pediatrics International, 60 (4), 372–375. https://doi.org/10.1111/ped.13519 . Epub 2018 Feb 28. PMID: 29337407.
Kudrnáčová, M., & Kudrnáč, A. (2023). Better sleep, better life? Testing the role of sleep on quality of life. PLoS One, 18 (3), e0282085. https://doi.org/10.1371/journal.pone.0282085 . PMID: 36920893; PMCID: PMC10016705.
Kundermann, B., Krieg, J. C., Schreiber, W., & Lautenbacher, S. (2004). The effect of sleep deprivation on pain. Pain Research and Management, 9 (1), 32.
Lanigar, S., & Bandyopadhyay, S. (2017). Sleep and epilepsy: A complex interplay. Missouri Medicine, 114 (6), 453–457.
Lee, K., Cho, M., Miaskowski, C., & Dodd, M. (2004). Impaired sleep and rhythms in persons with cancer. Sleep Medicine Reviews, 8 (3), 199–212.
Lucey, B. P., Hicks, T. J., McLeland, J. S., et al. (2018). Effect of sleep on overnight cerebrospinal fluid amyloid β kinetics. Annals of Neurology, 83 (1), 197–204.
Lumeng, J. C., Somashekar, D., Appugliese, D., Kaciroti, N., Corwyn, R. F., & Bradley, R. H. (2007). Shorter sleep duration is associated with increased risk for being overweight at ages 9 to 12 years. Pediatrics, 120 (5), 1020–1029.
Mallon, L., Broman, J. E., & Hetta, J. (2005). High incidence of diabetes in men with sleep complaints or short sleep duration: A 12-year follow-up study of a middle-aged population. Diabetes Care, 28 (11), 2762–2767.
McDermott, C. M., LaHoste, G. J., Chen, C., Musto, A., Bazan, N. G., & Magee, J. C. (2003). Sleep deprivation causes behavioral, synaptic, and membrane excitability alterations in hippocampal neurons. The Journal of Neuroscience, 23 (29), 9687–9695.
Meier-Ewert, H. K., Ridker, P. M., Rifai, N., et al. (2004). Effect of sleep loss on C-reactive protein, an inflammatory marker of cardiovascular risk. Journal of the American College of Cardiology, 43 (4), 678–683.
Mitter, P., De Crescenzo, F., Loo Yong Kee, K., Xia, J., Roberts, S., Chi, W., Kurtulmus, A., Kyle, S. D., Geddes, J. R., & Cipriani, A. (2022). Sleep deprivation as a treatment for major depressive episodes: A systematic review and meta-analysis. Sleep Medicine Reviews, 64 , 101647. https://doi.org/10.1016/j.smrv.2022.101647 . Epub 2022 May 26. PMID: 35700677.
Murck, H., Struttmann, T., Czisch, M., Wetter, T., Steiger, A., & Auer, D. P. (2002). Increase in amino acids in the pons after sleep deprivation: A pilot study using proton magnetic resonance spectroscopy. Neuropsychobiology, 45 (3), 120–123.
National Sleep Foundation. (2019, October). Why do we need sleep? https://www.sleepfoundation.org/articles/why-do-we-need-sleep
Nofzinger, E. A., Thase, M. E., Reynolds, C. F., 3rd, et al. (1991). Hypersomnia in bipolar depression: A comparison with narcolepsy using the multiple sleep latency test. The American Journal of Psychiatry, 148 , 1177–1181.
Novati, A., Hulshof, H. J., Koolhaas, J. M., Lucassen, P. J., & Meerlo, P. (2011). Chronic sleep restriction causes a decrease in hippocampal volume in adolescent rats, which is not explained by changes in glucocorticoid levels or neurogenesis. Neuroscience, 190 , 145–155.
Papantoniou, K., Massa, J., Devore, E., et al. (2019). Rotating night shift work and risk of multiple sclerosis in the Nurses’ Health Studies. Occupational and Environmental Medicine, 76 (10), 733–738.
Patel, S. R., Malhotra, A., White, D. P., Gottlieb, D. J., & Hu, F. B. (2006). Association between reduced sleep and weight gain in women. American Journal of Epidemiology, 164 (10), 947–954.
Philipsen, A., Hornyak, M., & Riemann, D. (2006). Sleep and sleep disorders in adults with attention deficit/hyperactivity disorder. Sleep Medicine Reviews, 10 , 399–405.
Ramanathan, A., Nelson, A. R., Sagare, A. P., & Zlokovic, B. V. (2015). Impaired vascular-mediated clearance of brain amyloid beta in Alzheimer’s disease: The role, regulation and restoration of LRP1. Frontiers in Aging Neuroscience, 7 , 136.
Rico-Rosillo, M. G., & Vega-Robledo, G. B. (2018). Sueño y sistema immune [Sleep and immune system]. Revista Alergia México, 65 (2), 160–170. https://doi.org/10.29262/ram.v65i2.359 . Spanish. PMID: 29983013.
Roy-Byrne, P. P., Uhde, T. W., & Post, R. M. (1986). Effects of one night’s sleep deprivation on mood and behavior in panic disorder. Patients with panic disorder compared with depressed patients and normal controls. Archives of General Psychiatry, 43 (9), 895–899.
Rudick, R. A., & Goelz, S. E. (2011). Beta-interferon for multiple sclerosis. Experimental Cell Research, 317 (9), 1301–1311.
Sabanayagam, C., Shankar, A., Buchwald, D., & Goins, R. T. (2011). Insomnia symptoms and cardiovascular disease among older American Indians: The Native Elder Care Study. Journal of Environmental and Public Health, 2011 , 964617. https://doi.org/10.1155/2011/964617
Sateia, M. J. (2014). International classification of sleep disorders-third edition: Highlights and modifications. Chest, 146 (5), 1387–1394. https://doi.org/10.1378/chest.14-0970 . PMID: 25367475.
Scheen, A. J., Byrne, M. M., Plat, L., Leproult, R., & Van Cauter, E. (1996). Relationships between sleep quality and glucose regulation in normal humans. American Journal of Physiology, 271 (2), E261–E270.
Sharma, S., & Kavuru, M. (2010). Sleep and metabolism: An overview. International Journal of Endocrinology, 2010 , 270832. https://doi.org/10.1155/2010/270832 . Epub 2010 Aug 2. PMID: 20811596; PMCID: PMC2929498.
Staniszewska, A., Mąka, A., Religioni, U., & Olejniczak, D. (2017). Sleep disturbances among patients with epilepsy. Neuropsychiatric Disease and Treatment, 13 , 1797–1803.
Steriade, M. (2005). Sleep, epilepsy and thalamic reticular inhibitory neurons. Trends in Neurosciences, 28 (6), 317–324.
Sung, V., Hiscock, H., Sciberras, E., & Efron, D. (2008). Sleep problems in children with attention-deficit/hyperactivity disorder: Prevalence and the effect on the child and family. Archives of Pediatrics & Adolescent Medicine, 162 , 336–342.
Taheri, S., Lin, L., Austin, D., Young, T., & Mignot, E. (2004). Short sleep duration is associated with reduced leptin, elevated ghrelin, and increased body mass index. PLoS Medicine, 1 (3), e62.
Vgontzas, A. N., Zoumakis, E., Bixler, E. O., et al. (2004). Adverse effects of modest sleep restriction on sleepiness, performance, and inflammatory cytokines. Journal of Clinical Endocrinology and Metabolism, 89 (5), 2119–2126.
Vgontzas, A. N., Liao, D., Pejovic, S., Calhoun, S., Karataraki, M., Basta, M., et al. (2010). Insomnia with short sleep duration and mortality: The Penn State cohort. Sleep, 33 (9), 1159–1164.
Wang, C., & Holtzman, D. M. (2020). Bidirectional relationship between sleep and Alzheimer’s disease: Role of amyloid, tau, and other factors. Neuropsychopharmacology, 45 (1), 104–120.
Yaggi, H. K., Araujo, A. B., & McKinlay, J. B. (2006). Sleep duration as a risk factor for the development of type 2 diabetes. Diabetes Care, 29 (3), 657–661.
Download references
Author information
Authors and affiliations.
Institute of Psychiatry, Faculty of Medicine, University of São Paulo, Sao Paulo, Brazil
Andrea Cecilia Toscanini
Institute of Psychiatry, Hospital das Clínicas, FMUSP, Sao Paulo, Brazil
You can also search for this author in PubMed Google Scholar
Corresponding author
Correspondence to Andrea Cecilia Toscanini .
Editor information
Editors and affiliations.
Department of Clinical Psychology, Universidade de São Paulo, São Paulo, São Paulo, Brazil
Renatha El Rafihi-Ferreira
Rights and permissions
Reprints and permissions
Copyright information
© 2024 The Author(s), under exclusive license to Springer Nature Switzerland AG
About this chapter
Toscanini, A.C., Hasan, R. (2024). Effects of Sleep Deprivation. In: El Rafihi-Ferreira, R. (eds) Acceptance and Commitment Therapy for Insomnia. Springer, Cham. https://doi.org/10.1007/978-3-031-50710-6_3
Download citation
DOI : https://doi.org/10.1007/978-3-031-50710-6_3
Published : 22 February 2024
Publisher Name : Springer, Cham
Print ISBN : 978-3-031-50709-0
Online ISBN : 978-3-031-50710-6
eBook Packages : Behavioral Science and Psychology Behavioral Science and Psychology (R0)
Share this chapter
Anyone you share the following link with will be able to read this content:
Sorry, a shareable link is not currently available for this article.
Provided by the Springer Nature SharedIt content-sharing initiative
- Publish with us
Policies and ethics
- Find a journal
- Track your research
Thank you for visiting nature.com. You are using a browser version with limited support for CSS. To obtain the best experience, we recommend you use a more up to date browser (or turn off compatibility mode in Internet Explorer). In the meantime, to ensure continued support, we are displaying the site without styles and JavaScript.
- View all journals
Sleep deprivation articles from across Nature Portfolio
Sleep deprivation is a state that arises when an organism has less sleep than is optimal, and is followed by a 'rebound' in slow-wave sleep when the opportunity arises. This can be induced experimentally or environmentally (such as shift work), but is also associated with neurological and psychiatric disorders such as dementia, in which normal sleep patterns are disrupted.
Latest Research and Reviews
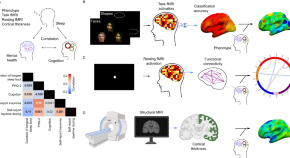
Opposing brain signatures of sleep in task-based and resting-state conditions
The associations between sleep, depression and brain activity are not well understood. Here, the authors show patterns of brain activity associated with insomnia and depression resemble those found in people who sleep less, but only under cognitive load. At rest, these activation patterns are hyperconnected and resemble those found in longer sleepers.
- Mohamed Abdelhack
- Peter Zhukovsky
- Daniel Felsky
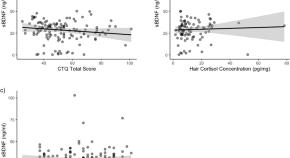
Interplay between stress, sleep, and BDNF in a high-risk sample of young adults
- Nimmy Varghese
- David Buergin
- Eva Unternaehrer
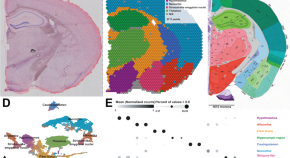
Spatial transcriptomics reveals unique gene expression changes in different brain regions after sleep deprivation
Sleep deprivation impacts molecular changes across brain regions. Here, the authors utilize a spatial. transcriptomics approach to elucidate acute sleep deprivation-induced gene expression signature. across regions and subregions of the brain.
- Yann Vanrobaeys
- Zeru J. Peterson
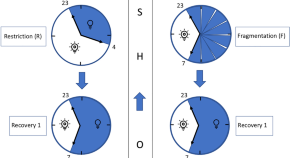

Effects of sleep fragmentation and partial sleep restriction on heart rate variability during night
- Julia Schlagintweit
- Naima Laharnar
- Ingo Fietze
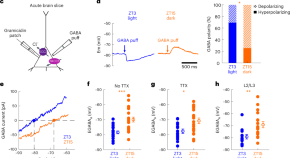
Intracellular chloride regulation mediates local sleep pressure in the cortex
Alfonsa et al. show that wakefulness causes shifts in cortical EGABA A , weakening synaptic inhibition and resulting in markers of local sleep pressure, and identify Cl − regulation as a link between sleep–wake history, cortical activity and behavior.
- Hannah Alfonsa
- Richard J. Burman
- Colin J. Akerman
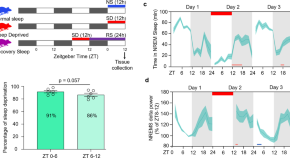
Single-cell transcriptomics and cell-specific proteomics reveals molecular signatures of sleep
Joint scRNA-seq and proteomic analysis of the hypothalamus, cortex, and brainstem in mice provides insight into the cellular and molecular underpinnings of sleep homeostasis.
- Pawan K. Jha
- Utham K. Valekunja
- Akhilesh B. Reddy
News and Comment
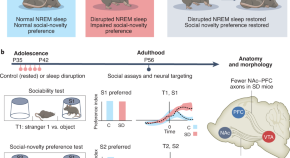
Adolescent sleep molds adult social preferences
Sleep disruption is a common but poorly understood feature of neurodevelopmental disorders including autism spectrum disorder. A study by Bian et al. reveals that sleep disruption in adolescent mice leads to long-lasting changes in social novelty preferences. Importantly, these perturbations can be restored through balanced actions in midbrain dopamine systems.
- Jenna A. McHenry
Shut eye and social bonds
- Ellen P. Neff
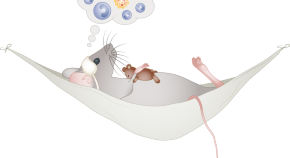
Sleep reduces haematopoiesis and atherosclerosis via a neuroimmune axis
Fragmented sleep interferes with a novel neuroimmune axis involving hypocretin produced in the hypothalamus, leading to increased haematopoiesis and larger atherosclerotic lesions.
- Gregory B. Lim
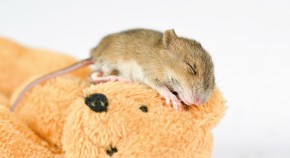
Sleep it off
A set of 80 (mostly synaptic) proteins show hyperphosphorylation in sleep-deprived mice and genetically ‘sleepy’ mice, suggesting that increased phosphorylation of such proteins may be associated with sleep need.
- Natasha Bray
I feel the need, the need for sleep
Sleep deprivation linked to blood–brain barrier disruption, quick links.
- Explore articles by subject
- Guide to authors
- Editorial policies


An official website of the United States government
The .gov means it’s official. Federal government websites often end in .gov or .mil. Before sharing sensitive information, make sure you’re on a federal government site.
The site is secure. The https:// ensures that you are connecting to the official website and that any information you provide is encrypted and transmitted securely.
- Publications
- Account settings
Preview improvements coming to the PMC website in October 2024. Learn More or Try it out now .
- Advanced Search
- Journal List
- HHS Author Manuscripts

The sleep-deprived human brain
Adam j. krause.
1 Department of Psychology, University of California, Berkeley
Eti Ben Simon
Bryce a. mander, stephanie m. greer.
2 Helen Wills Neuroscience Institute, University of California, Berkeley, California 94720–1650, USA
Jared M. Saletin
Andrea n. goldstein-piekarski, matthew p. walker.
How does a lack of sleep affect our brains? In contrast to the benefits of sleep, frameworks exploring the impact of sleep loss are relatively lacking. Importantly, the effects of sleep deprivation (SD) do not simply reflect the absence of sleep and the benefits attributed to it; rather, they reflect the consequences of several additional factors, including extended wakefulness. With a focus on neuroimaging studies, we review the consequences of SD on attention and working memory, positive and negative emotion, and hippocampal learning. We explore how this evidence informs our mechanistic understanding of the known changes in cognition and emotion associated with SD, and the insights it provides regarding clinical conditions associated with sleep disruption.
Without sleep, our cognitive and emotional abilities become markedly disrupted. What are the neural changes underlying these abnormalities? What do these alterations tell us about the pervasive link between sleep disruption and numerous neurological and psychiatric disorders?
There are at least three motivating reasons to build an accurate account of how sleep deprivation (SD) affects the human brain. First, from a neurobiological perspective, it is important to characterize which networks in the human brain are vulnerable or resilient to the effects of insufficient sleep and to understand how such SD-induced changes (such as regional or network increases or decreases in activity or changes in functional connectivity) explain the maladaptive changes in behaviour that are associated with SD. Of importance, SD does not simply represent the absence of sleep and the functions attributed to it. Rather, the sleep-deprived state is a composite of numerous detrimental factors, including extended wakefulness, as well as the absence of sleep. It is therefore insufficient only to develop an understanding of the functional benefits of sleep and then to reverse-infer an understanding of the neural and behavioural changes that would be expected following a lack thereof. Second, it is necessary to determine how comorbid sleep disruption — present in all major neurological and psychiatric conditions, including schizophrenia, Alzheimer disease, anxiety disorders and addiction disorders 1 , 2 — contributes to or results from these disorders and may thus be a target for disease treatment and/or prevention. Third, from a societal standpoint, such scientific evidence informs debates regarding sleep recommendations for both public and professional health policies in light of the acknowledged sleep-loss epidemic that now pervades industrialized nations 3 .
This Review seeks to move closer to these goals. We provide a focused overview of the impact of SD on the human brain across five functional domains: attention; working memory; positive, reward-related affect; negative affect; and hippocampus-dependent memory. Drawing on neuroimaging studies, we explore the neural signatures underlying phenotypic changes in cognition and affect following experimental SD. Moreover, we present examples of how these findings afford mechanistic insights into clinical disorders associated with disturbed sleep. The Review is necessarily focused on acute (24–48-hour) SD unless otherwise specified, as most neuroimaging studies to date are restricted to these time frames. Nevertheless, the issue of acute versus chronic sleep loss is discussed in more detail in BOX 1 .
Acute versus chronic sleep deprivation and inter-individual variability
A well-characterized feature of both acute sleep deprivation (SD) and chronic partial sleep restriction is a dose-dependent effect on task performance, especially in the domain of attention. The greater the amount and/or the longer duration of SD, the worse the accumulating attention deficit 6 . Thus, attentional impairment may be strongly coupled with extended wakefulness and with sleep pressure. These objectively measured impairments in performance are distinct from increases in subjective ratings of sleepiness following SD, which depend on the form of SD applied; for example, sleepiness increases as acute SD continues but does not always increase as chronic sleep restriction continues 5 . Thus, the physiological response to sleep loss may depend to some degree on the form of SD.
Regardless, the brain processes that mediate these cumulative deficits remain largely uncharacterized. For example, is there a brain region or set of brain regions involved in, or associated with, the tracking of sleep pressure 131 , or are there processes that track sleep pressure locally in a use-dependent manner within individual neuronal ensembles 132 ? Sleep pressure-related molecules, such as adenosine, and the hypothalamic system governing the switch mechanism between sleep and wake 133 are logical candidates for the chemical signalling and network mediation of this prototypical dose-dependent attentional impairment with sleep loss. In humans, neuroimaging analysis has revealed that association cortices, especially in the frontoparietal attention network, are particularly sensitive to sleep pressure, whereas subcortical thalamic and basal ganglia brain regions seem to be more affected by circadian processes 19 .
A second behavioural feature of SD that is poorly defined at the whole-brain level is a phenotypic difference in vulnerability to SD-associated cognitive impairments 134 – 138 . Approximately one third of participants show minimal impairments in sustained attention under SD conditions, and this seems to be a reliable, stable trait. By contrast, one third of participants manifest a severely vulnerable phenotype, showing marked attentional impairments. Moreover, it seems that differential vulnerability to the effects of acute sleep loss on attention is, in part, heritable 137 . Adding further complexity, the extent of an individual’s cognitive impairment also depends on which cognitive domain is tested 130 . Thus, although performance impairments following SD within any given cognitive domain are highly reliable within the same individual, the degree of impairment is not necessarily reliable across cognitive domains within the same individual. The underlying brain correlates that differentiate these stable intra-individual and inter-individual differences are unclear, but the functional integrity of the frontoparietal attention network and genetic influences on sleep and circadian regulation are probably key factors 139 , 140 .
Attention and working memory
One cognitive ability that is especially susceptible to sleep loss is attention, which serves ongoing goal-directed behaviour 4 . Performance on attentional tasks deteriorates in a dose-dependent manner with the amount of accrued time awake, owing to increasing sleep pressure 5 – 7 . The prototypic impairments on such tasks are known as ‘lapses’ or ‘microsleeps’, which involve response failures that reflect errors of omission 4 – 6 . More specifically, attentional maintenance becomes highly variable and erratic (with attention being sustained, lost, reestablished, then lost again), resulting in unstable task performance 4 . Although the focus of this Review is sleep loss, it should be noted that daytime circadian alerting signals interact with SD, resulting in exponentially scaling attentional impairment with extended wakefulness (for reviews, see REFS 7 , 8 ). Nevertheless, the cumulative amount of extended time spent awake predicts lapses in attention with acute SD and with chronic partial sleep restriction (the latter occurring across many nights) 5 ( BOX 1 ). Why some individuals are more or less vulnerable to these attentional impairments following SD than others is less well understood.
An increasingly clear picture is emerging of how brain function related to attentional tasks is altered by acute SD. Reductions in functional MRI (fMRI) signal in the dorsolateral prefrontal cortex (DLPFC) and intraparietal sulcus while performing attentional tasks are a robust and reliable consequence of SD 9 – 16 ( FIG. 1 ). Indeed, sleep loss not only decreases task-related activity in these frontal and parietal regions but also diminishes activity in, and connectivity with, the extrastriate visual cortex during visuospatial attention tasks 9 – 11 . The behavioural consequences of these neural changes are reflected in deficiencies in attending to one specific stimulus while ignoring distractors 10 , 11 , 17 or deficits in top-down allocation of attentional resources, such as orienting to a location where a target is expected to appear 9 , 18 . Beyond attentional focus at any particular moment, sleep loss impairs the capacity to sustain attention over time 4 . Here again, reductions in activity in the DLPFC and intraparietal sulcus contribute to attentional performance failure 11 – 15 , 19 .

a | Brain regions and networks associated with attention and working memory (frontoparietal network (FPN); red), arousal (thalamus; green) and the default mode network (DMN; blue) are affected by sleep deprivation. The DMN is a collection of brain areas, including midline frontoparietal regions, that often disengage when an individual performs an externally driven, goal-directed task and then re-engage when an individual stops performing that task. Suppression of the DMN is necessary to mobilize appropriate on-task brain networks to achieve successful goal-directed behaviour 181 , unless that task requires access to internally stored relevant information such as autobiographical memories or previously learned predictive cues 18 , 182 , 183 . Brain regions across multiple brain networks, including the dorsolateral prefrontal cortex (DLPFC), intraparietal sulcus (IPS), thalamus, medial prefrontal cortex (mPFC) and posterior cingulate cortex (PCC), are differentially altered by sleep loss. b | In the sleep-rested state, there is reciprocal inhibition between task-related FPN activity and DMN activity, supported by sustained ascending arousal input from the thalamus. This leads to consistent attentional and working-memory performance. In the sleep-deprived state, there is unstable reciprocal inhibition between task-related FPN activity and DMN activity, and erratic ascending arousal activity influencing thalamic activity. As a result, there is reduced task-related FPN activity and intermittent intrusions of DMN activity during task engagement. The behavioural consequence is variable and/or impaired attention and working-memory performance, worsening with lowered thalamic activity and improving with higher thalamic activity.
In addition, thalamic activity during sustained attention is altered with SD, suggesting that the thalamus could be an interacting node in this SD-affected network. However, the profile of activity change within the thalamus is not uniform. Some studies demonstrated greater activity under conditions of sleep loss 11 , 16 , 20 – 23 , whereas others have reported intermittent periods of diminished thalamic activity 10 , 12 . These discrepancies may be understood in the context of performance and of the cortical arousal that thalamic activity provides. When thalamic activity is elevated under conditions of sleep loss, attentional performance is frequently maintained but, when substantial reductions in thalamic activity are observed, lapses in attention are common 4 , 10 , 12 , 15 . The thalamus therefore represents a pivotal gating hub through which alterations in brainstem ascending arousal signals affect cortical attentional networks under SD conditions. Extant data therefore support a model in which the instability in ascending arousal-promoting input contributes both to the emergence of canonical attentional lapses during SD and the erratic, unpredictable expression of these lapses over time 4 , 24 ( FIG. 1 ). Notably, the observed reductions in thalamic activity are not present during attentional lapses in well-rested conditions 12 , suggesting that increased homeostatic sleep pressure may have a unique role in lapses following SD.
More recently, instability of the default mode network (DMN) has been implicated in attentional impairments with SD. Several reports describe an inability to fully disengage midline anterior and posterior cortical regions of the DMN during both selective and sustained attentional task performance under SD conditions 13 , 16 , 25 ( BOX 2 ; FIG. 1 ). Moreover, increased DMN activity during on-task performance for both sustained and selective attention tests was predictive of slower and less accurate performance by the participants 16 , 25 .
The unrested resting brain: sleep loss and resting-state activity
In addition to inducing differences in task-related brain activity, sleep deprivation (SD) also affects resting-state brain connectivity. Resting-state networks are typically derived from the connectivity profile of spontaneous fluctuations in functional MRI (fMRI) signal and are thought to reflect the brain’s intrinsic functional architecture that emerges without external task demands 141 , 142 . Across these networks, SD is associated with reduced connectivity within the default mode network (DMN), the dorsal attention network, and the auditory, visual and motor networks 143 – 145 . In fact, measures of abnormal change in whole-brain connectivity enable classification of an individual as either being rested or sleep deprived with more than 60% accuracy 144 .
Of the many different discrete resting-state networks, the DMN has demonstrated alterations under SD in two specific ways. First, connectivity between individual nodes of the DMN is decreased following one night of total SD 143 , especially between midline anterior and posterior nodes of the DMN 146 . Thus, SD degrades network integrity within the DMN, functionally uncoupling individual nodes. The second consequence of SD is the failure of the DMN to remain functionally distinct from other normally dissociable networks. Under normal rested conditions, the DMN is functionally decoupled from the brain’s attentional networks — when the latter is engaged, the former is disengaged, and vice versa 147 . Both total SD and partial sleep restriction 122 , 148 – 150 impair this adaptive decoupling, and this impairment is further associated with participants’ cognitive vulnerability to SD 143 .
The resting profiles of individual regions, specifically the amygdala and the thalamus, have also been examined following SD 151 , 152 . Similar to task-related connectivity changes (see FIG. 3 ), decreases in resting-state connectivity of the amygdala with regulatory and executive regions of the dorsolateral prefrontal cortex and anterior cingulate cortex have been demonstrated after 36 hours of SD. Conversely, resting-state connectivity of the amygdala with the posterior cingulate cortex and the precuneus increases after SD, possibly reflecting the role of these posterior midline cortical regions in regulating the balance between internally and externally directed cognition 153 . Resting-state connectivity between the thalamus and cortical regions is also generally decreased following sleep loss, especially thalamic connectivity with anterior and posterior cingulate regions of the DMN 143 and with attentional and executive regions of the superior and medial prefrontal cortex 151 . As reductions in thalamocortical connectivity have also been demonstrated during sleep onset 154 , it remains possible that resting-state measures in sleep-deprived individuals capture mixed states of sleep and wake, comprising rapid transitions into sleep (known as microsleeps), while some degree of wake-like behavioural responding is nevertheless maintained 155 . This concern is emphasized by reports that have demonstrated reduced functional coupling between midline anterior and posterior nodes of the DMN under conditions of SD and once individuals have entered non-rapid eye movement sleep 156 . Overall, the limited evidence to date suggests that SD triggers reductions in the brain’s intrinsic connectivity profile, ultimately leading to a breakdown of network integrity and a loss of adaptive functional segregation. These alterations may precede or exacerbate changes in task-related responses observed after sleep loss, and might predict the magnitude of cognitive and emotional impairments elicited by SD. A combination of task-related and resting-state fMRI studies of SD seems to be a fruitful avenue for future work, although accurate assessment of sleep or wake states inside the MRI scanner is warranted to assure the absence of microsleeps.

a | Sleep deprivation (SD) amplifies amygdala reactivity (red) in response to negative emotional stimuli and decreases associated amygdala–medial prefrontal cortex (mPFC) connectivity (blue). b | SD alters sensitivity of the salience-detection network (the amygdala, anterior cingulate cortex (ACC) and anterior insula (AI)) to varying levels of emotional stimuli that range in valence strength ( x axis in the two graphs) from negative (red) to neutral (blue) to positive (red). Under sleep-rested conditions (left graph), there is a wide and dynamic range of salience-detection sensitivity, resulting in the ability to accurately discriminate between degrees of emotional salience (tall vertical difference arrow; discerning emotional (red vertical line) from neutral stimuli (blue vertical line)). By contrast, SD (right graph) is proposed to trigger a narrowing and thus more nonspecific detection sensitivity range, impairing the ability to accurately discriminate between degrees of emotional salience (short vertical difference arrow). This loss of ‘net neutrality’ results in over-generalized emotional sensitivity, wherein an otherwise largely neutral stimulus (blue vertical line) is inappropriately registered as ‘emotional’ by the salience-detection network; further observed in biased emotional ratings of neutral stimuli. c | A downstream behavioural consequence of these central brain changes, in combination with disrupted peripheral autonomic nervous system feedback of visceral body information, could lead to inaccurate and even absent outward expression of emotions. This is supported by experimental evidence demonstrating that individuals deprived of sleep fail to register emotional faces shown to them on a screen and consequently are unable to accurately mimic the emotional face expressions of these target faces themselves. Part a is adapted with permission from REF. 81 , Elsevier.
Why the sleep-deprived brain suffers this unstable ‘flip-flop’ gating between on-task functional activity and off-task DMN activity remains unclear. One candidate is abnormal activity of the right frontoinsular cortex, which controls the switch between DMN activity and task-related activity 26 . Consistent with this thesis, the salience-detection network, which includes the frontoinsular cortex, demonstrates reduced activity during the performance of attention tasks following sleep loss 27 . Together with changes in the frontoparietal attention network described above, these insula-based alterations may explain SD-induced impairments in attention to novel, salient targets 28 and in the tracking of salient moving targets 29 . Nevertheless, trial-by-trial analyses of fMRI signal during salience-detection and attention tasks will be required to test this hypothesis.
Working memory
Working memory — the neural basis of which overlaps anatomically with the attention system — is also impaired by SD 30 , 31 . Deficits in both working- memory and attention tasks have been found to correlate with reductions in DLPFC and posterior parietal activity 20 – 22 , 32 , 33 . As during attention tasks, fluctuations in thalamic activity 20 – 22 and inappropriate perseverance of DMN activity are observed during working-memory task performance under SD conditions 20 , 21 , 32 . In addition, the degree of aberrant, on-task DMN activity predicts the severity of working-memory impairment in sleep-deprived individuals 20 , 21 , 32 ( FIG. 1 ). Inappropriate gating of on-task relative to off-task network control may therefore provide one common mechanism underlying deficits in both attention and working memory caused by SD.
Also mirroring changes observed during attention tasks, alterations in thalamic activity and connectivity predict impairments in working-memory performance under SD conditions, most likely owing to the key role of the thalamus in cortical arousal. For example, increased connectivity between the hippocampus, the thalamus and the DMN predicts higher subjective sleepiness and is associated with worse working-memory performance under sleep-loss conditions 34 , 35 . By contrast, increased connectivity between the thalamus and the precuneus under SD conditions predicts greater recovery of working-memory performance, relative to the sleep-rested state. This finding is consistent with the hypothesis that, in the context of SD, the brain exhibits compensatory neural activity that can enable partial recovery of the performance of certain behaviours 34 , 36 .
Beyond reductions in activity in frontoparietal areas, extrastriate (visual) cortical regions also demonstrate reduced signal during visual working-memory task performance under conditions of sleep loss 21 , 22 , 32 . Recent evidence suggests a causal contribution of this regional decrease in task-related activation to performance deficits, as transcranial magnetic stimulation (TMS) targeted to the extrastriate cortex of sleep-deprived individuals improved visual working-memory performance, restoring it back to baseline levels 37 , 38 . Remarkably, repeated TMS application every 6 hours for 18 hours to extrastriate regions maintained working-memory performance for up to 3 days without sleep 38 . However, TMS stimulation intervention in the context of SD is not always consistent, and thus the reproducibility of such effects remains unclear 39 . Should a robust method for intervention emerge, it may have real-world relevance — for example, for professions in which attentive focus is crucial and SD is common (aviation or the military).
Reward and incentive processing
Reward processing in the sleep-deprived brain.
The mesolimbic reward system is a network of interconnected brain regions, including the midbrain ventral tegmental area, striatum and regions of the PFC. The ventral tegmental area provides dopaminergic innervation to the striatum, which is connected to, and regulated by, areas of the PFC, particularly by the medial PFC (mPFC) and inferior orbitofrontal cortex (OFC) regions, guiding motivated actions and learning. This system has repeatedly been demonstrated to show sensitivity to SD, leading to alterations in motivated behaviours, such as risk taking, sensation seeking and impulsivity ( FIG. 2 ).

a | Reward-relevant brain regions that are affected by sleep deprivation (SD) include cortical regions (blue) such as the medial prefrontal cortex (mPFC), insula and orbitofrontal cortex (OFC), and the subcortical region of the striatum (red). b | Increased adenosine load (blue circles) associated with SD triggers downregulation of dopamine (DA) D2 and D3 receptors (D2/3Rs), resulting in decreased receptor membrane expression within the striatum (internalized receptors; grey). Consequently, there is a greater ratio of D1R to D2/3R availability, and the relative increase in striatal D1R activation by DA (green circles) under SD conditions is proposed to increase risk-related and reward-related approach behaviours, shown in the see-saw imbalance. c | SD further disrupts incentive processing within PFC regions and thus is proposed to result in a lower signal-to-noise ratio (SNR) under SD conditions (represented by a narrow dynamic range of PFC sensitivity). This consequentially impairs the ability to accurately map and update changing value or reward probability (coloured dots) over time — such as that involved in reinforcement learning, ultimately contributing to non-optimal incentive-based decision making.
In rats, SD disrupts dopaminergic function by modifying dopamine receptor sensitivity and availability in basal ganglia regions 40 , 41 . Humans deprived of sleep for one night show increases in ventral striatum activity in mixed monetary gamble task during the anticipation and receipt of monetary rewards 42 , 43 . Activity in affect-related regions in the frontal cortex that are associated with valuation and viscerosensory functions, including the insula and mPFC, is also substantially increased following SD 42 , 43 .
Together, these findings suggest that subcortical reward-related regions of the brain, together with related cortical regions coding salience and valuation, seem to become hypersensitized by the state of acute SD. However, the degree to which fMRI signal amplitude tracks reward magnitude in these subcortical and cortical regions does not seem to differ between rested and deprived conditions 44 . Rather, SD triggers a generalized increase in reward sensitivity that impairs reward discrimination accuracy, such that the brain becomes less capable of accurately coding incremental increases in reward value, from low to high.
Consistent with this thesis, fMRI signal in the mPFC, OFC and anterior insula cortex in sleep-deprived individuals does not accurately discriminate between trials involving monetary reward and punishment values and trials involving no monetary reward or punishment 42 , 43 . This inaccurate representation of reward value within frontal regions is similarly observed during the outcome phase of incentive decision trials 42 , 43 , suggesting a further failure to update accruing reward history and probability. Inaccurate coding of reward and/or punishment valence in the PFC following sleep loss may therefore prevent the ability to update changing incentive value (weights) of reinforcing stimuli over time 42 , 43 ( FIG. 2 ). This would further contribute to non-optimal reward-dependent decision making and actions. Fitting this profile, sleep-deprived individuals performing the Iowa Gambling Task make more-risky decisions and assign greater weights to more-recent rewards 45 , 46 . This pattern indicates a failure to appropriately integrate rewards and their accumulating value over time following SD, reflecting temporally shorter -sighted updating of rewards.
In addition to deficits in the activation of frontal reward circuits following SD, studies have revealed that responses of the striatum and amygdala to emotionally pleasurable or hedonic images 47 , as well as desirable food stimuli 48 , are amplified with SD. Moreover, the same studies reported failures of discriminatory signalling of stimulus valence (for example, discriminating desirable from undesirable foods) in the anterior insula, mPFC and OFC, of sleep-deprived participants. Furthermore, sleep- deprived individuals were more likely to rate neutral pictures as positive 47 or to express a greater desire for high-calorie foods 48 . That is, participants fail to accurately disambiguate non-rewarding from rewarding stimuli when sleep deprived, shifting towards an over-generalized reward bias, even for normally less-rewarding stimuli.
Although current results of reward processing point to effects in the mPFC, OFC and insular cortex, together with the basal ganglia, other affective regions, such as the limbic regions of the medial temporal lobe and executive lateral regions of the PFC, should not be discounted. Equally important, not all human SD studies have reported amplified basal ganglia activity during reward-related decision making 49 , an inconsistency we return to below.
Beyond monetary reward processing, another behavioural feature sensitive to a lack of sleep is impulsivity. Impulsivity is a multifactorial construct, and different types of impulsivity can manifest in behaviourally different ways. Nevertheless, impulse control is central to many incentive-based decision processes 50 , 51 . Changes in impulsivity may therefore contribute to alterations in reward decision making and risk taking that occur following SD.
In tasks that require either cued motor response execution or withholding, often referred to as a Go/No-Go task, participants with either partial sleep restriction (6 hours of sleep per night for 4 consecutive nights) or one night of total sleep deprivation showed considerably lower response inhibition and cognitive control, produced more frequent errors of inhibition, and exhibited slower learning of cue–incentive associations 52 – 56 ( FIG. 2 ).
However, in delay-discounting tasks, which require participants to choose between small, immediate rewards relative to large, delayed rewards, SD does not seem to alter impulsivity — a lack of an effect that is similarly observed in probabilistic discounting tasks, in which participants choose between small but certain rewards and large but uncertain rewards 49 , 53 , 54 , 57 . Similarly, total acute SD and partial sleep restriction (4 nights of 6 hours of sleep per night) do not affect delay discounting or probabilistic discounting 54 . However, measures of effort discounting (whereby rewards are discounted according to the effort required to attain them) are affected by SD. Under conditions of sleep loss, low-effort rewards elicit even greater reward-related brain activity than high-effort rewards, relative to sleep-rested conditions 49 .
Similarly, inconsistent effects of SD have been reported using the Balloon Analogue Risk Task (BART) — a standard test of reward-seeking, risk-taking behaviour that involves impulse control. Partial sleep restriction (1 night of 5 hours of sleep) results in a significant increase in the tendency to overinflate a computerized balloon to obtain more reward 54 , 58 . By contrast, total SD for a single night resulted in no change in BART performance by male participants but a decrease in risky overinflation by female participants 53 (for a review, see REF. 59 ). Studies that focus on this and other specific features of impulsivity, such as response withholding, while systematically keeping other factors constant (for example, reward magnitude, reward delay and sex) are necessary to develop a clearer understanding of sleep loss and risk-taking impulsivity.
Inconsistencies in the effects of SD on incentive processing are not limited to the domain of impulsivity. Some reports using incentive tasks that do not involve impulsivity (wherein participants simply expect or receive rewards and/or punishments without needing to resist impulsive responses) have also failed to show significant differences in mesolimbic brain activity and/ or behaviour caused by SD 44 , 49 , 57 . Instead, these studies only demonstrate effects on an individual-participant level 44 , 49 , 57 . One possible explanation is that some studies employ mixed gambles, combining gains and losses in the same trial. This may limit the ability to identify differences in processing of gains or losses independently 60 . A second possibility is that individual differences may interact with the effects of SD, thus obscuring group-level significance if not explicitly considered. A recent study 60 found that individuals expressing a variant of the dopamine transporter that was previously associated with differences in synaptic dopamine availability showed different striatal reward responses following sleep loss. Unlike the control group, only participants with trait-elevated synaptic dopamine exhibited greater striatal responses during reward anticipation following SD than when well rested. This suggests that phasic dopamine availability is increased in these individuals, thereby elevating SD-associated increases in the reward responsivity of the nucleus accumbens. Thus, effects of sleep loss on reward processing seem to be sensitive to several interacting factors, including sex and trait genetics.
Sleep deprivation and dopamine function
With these nuances taken into account, studies to date nevertheless indicate that SD significantly increases the tendency of reward sensitivity, risk taking and impulsivity, and disrupts reward-value updating and integration. One possible mechanism that might explain these increases in approach and consummatory behaviour is altered dopamine signalling, which is associated with extended wakefulness perhaps more than with the absence of sleep itself. Several lines of evidence support this dopaminergic framework ( FIG. 2 ).
First, dopamine is associated with arousal; innately higher levels of dopamine predict lower sleep propensity 61 . Second, rodent studies have established that wake-promoting stimulant drugs such as amphetamine seem to operate in part by blocking dopamine metabolism, thereby increasing dopamine transmission and thus arousal. Third, depleting catecholamines, including dopamine, reduces wake propensity, lowering vigilance and inducing sleep 62 (although interestingly the antihypertensive and antipsychotic reserpine, which reduces catecholamine function, has nominal effects on sleepiness 63 ).
Changes in dopamine receptors may also contribute to alterations in reward-driven behaviour following SD. Positron emission tomography (PET) ligand studies have reported that one night of total SD downregulates the availability of dopamine D2 and D3 receptors (D2/3Rs) in both the dorsal striatum (which includes the caudate and putamen) and ventral striatum 40 ( FIG. 2 ), consistent with earlier PET studies showing general hypometabolism in the basal ganglia following SD 64 . PET-assessed downregulation of D2/3Rs in the ventral striatum predicted fMRI-measured decreases in thalamic activity during an attentional task 65 , as did the degree of generalized hypometabolism in the basal ganglia after SD 64 , 66 . Besides the relevance of this change for objective attention and working -memory performance, the degree of D2/3R downregulation similarly predicts subjective sleepiness under SD conditions 40 . This result suggests that sleep pressure might be indexed by the levels of different dopamine receptors; consistent with this, adenosine accumulates with increasing time awake, activating A 2A receptors and thereby driving D2R internalization 40 , 67 . Moreover, agonists of the A 2A receptor and D2R demonstrate allosteric interaction such that A 2A receptor agonists decrease the affinity of D2Rs for their agonists, including dopamine 68 . Thus, adenosine accumulation, which is associated with increased time spent awake, may decrease D2/3R activity by at least two possible mechanisms: by increasing D2/3R internalization and by reducing binding of dopamine to D2Rs ( FIG. 2 ).
That SD is associated with a downregulation of D2/3Rs may initially seem to contradict the SD-associated increases in neural and behavioural reward sensitivity described above. However, one tenable (and experimentally testable) hypothesis that may reconcile this paradox involves D1Rs. Specifically, decreases in D2/3Rs could result in an imbalance of dopamine receptor availability, leading to the remaining D1Rs becoming disproportionately stimulated by the same amount of presynaptically released dopamine ( FIG. 2 ). Outside the context of SD, in rodents, this imbalance causes greater approach behaviour to food rewards 69 and increases the addictive potential of cocaine 70 (behavioural consequences that, notably, have both been independently associated with SD 71 , 72 ). Furthermore, the increase in striatal fMRI signal elicited by reward incentives under SD conditions seems to principally reflect D1R action 73 . Thus, SD-associated decreases in D2/3R availability may, by indirectly increasing dopamine binding to the remaining D1Rs, increase approach-driven and reward-driven behaviour and fMRI-indexed striatal activity ( FIG. 2 ). The neural and behavioural consequences of sleep loss on incentive processes also offer mechanistic and therapeutic insights into the conditions of obesity, addiction and substance use ( BOX 3 ).
Clinical insights concerning sleep loss and emotion
Positive emotion
The interaction between sleep deprivation (SD) and dopaminergic reward functioning is relevant for clinical conditions involving sleep disruption and dysfunction of reward or dopamine signalling. In alcohol addiction, ~60% of alcohol-addicted individuals seeking treatment complain of insomnia symptoms in the 6 months leading up to intervention, and more than half of these individuals report using alcohol under the (erroneous) assumption that it helps to regulate sleep 157 . In addition, alcohol-addicted individuals who report insomnia are more likely to relapse than those without sleep problems 157 . Thus, sleep loss seems to be an important factor in the development and/or maintenance of addiction disorders associated with changes in mesolimbic dopamine function and a vulnerability factor predisposing individuals to fail in efforts of abstinence. Of concern, sleep disruption in childhood predicts subsequent substance use in later adolescence stages 158 .
There is now also considerable epidemiological evidence linking sleep disruption and obesity 159 . Experimental work has characterized the associated causal mechanisms, including changes in the appetite-regulating hormones leptin and ghrelin, and in the central brain mechanisms that regulate appetite and food choice selection 160 . Decreased activity in decision-regulating regions of the frontal cortex following SD, combined with enhanced subcortical mesolimbic sensitivity, leads to increased desire for, and selection of, high-calorie food items 48 . Indeed, compared with a sleep-rested state, experimental sleep restriction increases calorie consumption and promotes weight gain 161 .
Notably, the alterations in brain function that have been associated with obesity overlap with those observed in drug addiction 162 ; for example, a reduction of dopamine D2 and D3 receptors is observed in both. Such findings warrant a deeper consideration of insufficient sleep as a possible predisposing factor underlying obesity and drug addiction. If true, targeted sleep restoration may aid by re-engaging neural mechanisms that govern adaptive food choices and craving, respectively.
Negative emotion
A recent model has proposed that sleep loss prevents the normal overnight lowering and thus restoration of central adrenergic signalling that normally occurs during rapid eye movement (REM) sleep, leading to heightened noradrenergic tone and thereby an over-generalized responsivity within select affective salience networks 84 . The translational ramifications of this model are well exemplified in post-traumatic stress disorder (PTSD), which is associated with sleep disruption and fragmentation, decreases in total and REM sleep duration 163 – 165 , and nocturnal hyperarousal 166 – 168 . In parallel, people with PTSD show marked alterations in noradrenergic activity 163 , including the lack of normal overnight reduction in noradrenaline levels 169 . The REM-sleep model may help to understand these co-occurring features. Specifically, decreases in night-time, total and REM sleep quantity, and increases in high-frequency electroencephalogram (EEG)-assessed activity (associated with hyperarousal) during REM sleep, are proposed to contribute to daytime hyperadrenergic tone in PTSD. Moreover, persistent hyperadrenergic activity during wakefulness could reduce subsequent night-time sleep quantity and quality, producing a vicious cycle. Crucially, this cycle could lead to over-generalized emotional sensitivity within the affective salience network of the brain that is innervated by noradrenaline, including the amygdala, and the viscerosensory regions of the insula and anterior cingulate cortex, as already reported in a meta-analysis of functional MRI studies in PTSD 89 . This same model could explain how nocturnal use of prazosin — an α1-adrenergic receptor antagonist commonly prescribed for PTSD — can, in some cohorts, restore the duration and quality of total and REM sleep, and decrease PTSD symptoms 170 – 173 . Our framework may also account for the excessive emotion reactivity and impaired emotional discrimination observed in PTSD 174 – 176 , and the maladaptive generalization of fear responses to commonly non-threatening stimuli 174 – 176 . Interestingly, PTSD-like symptomatology was experimentally induced by selective deprivation of REM sleep in healthy individuals; this manipulation resulted in increased autonomic sensitivity to previously extinguished conditioned stimuli (that is, heightened sensitivity) and impaired autonomic discrimination of conditioned from safety signals (decreased emotional specificity) 177 – 179 . Conversely, the amount and EEG quality of REM sleep predicts participants’ ability to accurately discriminate between threat and safety signals (indicating improved emotional specificity) 180 .
Aversive stimulus processing
Sleep loss reliably triggers changes in negative (aversive) emotional processing, including irritability, emotional volatility, anxiety and aggression 56 , 74 – 77 , as well as suicidal ideation, suicide attempts and suicide completion 78 – 80 . These findings suggest that SD alters specific process domains, including basic affective reactivity, as well as emotional discrimination and emotional expression, which are more complex.
Aversive stimulus response
Early neuroimaging studies focused on the effects of SD on responsivity to rudimentary aversive stimuli; for example, one night of SD was shown to result in a 60% increase in amygdala reactivity to negative images, such as weapons, venomous snakes and spiders, and mutilations 81 ( FIG. 3 ). This signature of amygdala hyper-reactivity has since been replicated following sleep restriction (4 hours of sleep per night for 5 nights) 82 and in individuals with poor sleep quality, as assessed at their homes 83 . Notably, amygdala hyper-reactivity has been reported during rapid, subconscious viewing of faces expressing fear after sleep restriction 82 , indicating that this amplified limbic reactivity can occur subliminally, independent of conscious, deliberative cognition 82 .
One possible mechanism underlying such amygdala hypersensitivity involves a loss of regulatory control, resulting in contextually inappropriate amygdala reactivity 84 . Decreases in functional connectivity between top-down control regions of the mPFC and the amygdala have been reported following a night of total SD or 5 nights of partial sleep restriction (4 hours sleep per night), as well as in participants reporting less than 6 hours of habitual sleep 81 – 83 , 85 , 86 ( FIG. 3 ).
SD further alters the brain anticipation of cued emotional experiences. For example, one night of SD elevates cue-evoked activity in the amygdala, anterior insula and anterior cingulate cortex in anticipation of impending emotional picture slides, similar to those described above 87 , and also increases anticipatory responses of the peripheral autonomic nervous system 88 .
Interestingly, inter-individual differences in trait anxiety levels predict the size of these SD-induced increases in anticipatory brain activity, with highly trait-anxious individuals expressing the largest increases in pre-emptive anterior insula activity 87 . The latter result is of clinical relevance to anxiety disorders (which have been associated with apprehensive worry about future events) for at least three reasons: first, highly trait-anxious individuals are already those at greatest risk for developing an anxiety disorder; second, excessive anticipatory insula activity is a characteristic of most anxiety disorders 89 – 91 ; and third, sleep disruption is a recognized symptom of anxiety disorders 92 .
Emotion discrimination and expression
An additional phenotype of the sleep-deprived human brain emerges when participants are challenged with the more complex task of disambiguating between varied emotional signals. In sleep-rested participants, regions of the amygdala, insula and cingulate that are associated with salience detection can discriminate between stimuli of different emotional strengths. By contrast, in sleep-deprived participants, these regions demonstrate a saturated and flattened response to emotional stimuli. Sleep-deprived individuals therefore express a generalized excess of emotional sensitivity, with impairment in emotional discriminatory specificity 84 ( FIG. 3 ). For example, sleep-deprived individuals are less accurate at rating facial expressions within the moderate range of emotional strength 93 and rate neutral images as more emotionally negative 94 . SD further impairs the accurate discrimination of threatening (antisocial) from affiliative (pro-social) facial signals 95 , again promoting an overall bias towards increased (inaccurate) perception of negative threat.
Recent work has begun to uncover the neural basis of these impairments in emotional discrimination ( FIG. 3 ). Consistent with the failure to accurately code positive, reward incentive signals (described above), one night of SD impairs the discrimination of faces expressing negative emotions by viscerosensory regions of the anterior insula and anterior cingulate cortices, and to a degree, the subcortical amygdala 95 . Similarly, sleep loss results in generalized, nonspecific increases in amygdala activity (as measured by fMRI or electroencephalography) in response to aversive and neutral emotional pictures 96 , 97 .
These findings have led to the proposal that SD causes a state of central and peripheral emotional hypersensitivity that prevents emotional reactivity from being appropriately graded 84 . As a result, SD impairs the afferent–efferent communication between the brain and body, which is crucial for the ‘embodied’ mapping of, and disambiguation between, different emotions. The consequence is a behavioural phenotype of indiscriminate emotional generalization, or blunting, consistent with poor signal-to-noise balance during emotional processing 84 ( FIG. 3 ).
Such blunting may disrupt not only the internal mapping of an individual’s own affective state but also the ability to simulate the feelings of others. Supporting this hypothesis, sleep-deprived individuals and habitually poor sleepers demonstrate impaired empathetic sensitivity, which involved sensing the emotion state of individuals in picture slides 98 , 99 . Sensing the internal homeostatic status of the body, and thereby tracking one’s own affective state, requires interoceptive processing within a network that includes the insula, mPFC and amygdala 100 . Sleep loss degrades the sensitivity of this network, leading to a shift of the homeostatic set point used to register, and differentiate between different levels of, emotional salience 95 . In an emotional face discrimination task, sleep-deprived individuals showed overgeneralized activity in viscerosensory brain regions and impaired autonomic cardiac discrimination (indexed using moment-by-moment changes in heart rate) 95 . Compared with when they were rested, individuals who were sleep deprived also showed a weaker correlation between the activity of the central and peripheral autonomic (cardiac) systems. Thus, SD compromises the faithful discrimination of emotional signals within higher-order emotional brain regions by inducing network hypersensitivity and, in tandem, disrupts the ‘embodied’ reciprocity between viscerosensory central and peripheral autonomic processing of complex social signals. As a result, there can be a loss of sensitivity in detecting, and accuracy in recognizing, emotions.
It is possible, although currently untested, that this same disruption within and between central and peripheral autonomic nervous system networks explains why sleep-deprived adults 101 and infants deprived of a day-time nap 102 show incongruent or even absent outward expression of emotions ( FIG. 3 ). Individuals restricted to 4 hours of sleep for a single night also demonstrate a slowing of reactive facial expressions in response to emotional stimuli 103 . Emotional vocalizations following short-term sleep restriction have similarly been reported to be blunted 104 .
Thus, multiple modes of emotional expression are diminished by insufficient sleep; a feature pertinent considering that emotional expressions are all influenced in some manner by the autonomic nervous system, specifically the vagal system 105 . This same explanatory model of central–peripheral autonomic decoupling may offer further mechanistic insights into the recognized associations between reduced sleep and decreased interpersonal functioning 108 , increased antisocial interactions 109 , a lower understanding of relationship concerns (leading to greater interpersonal conflict) 110 and, in children and adolescents, increased peer-related problems, such as hyperactivity and inattention, conduct problems, and peer disagreement 106 , 107 .
Less understood are the cellular and molecular mechanisms underlying the emotional brain and body dysfunction that is caused by a lack of sleep. One proposed mechanism has centred on the heightened noradrenergic tone from the locus coeruleus 84 , 111 , 112 ; according to this hypothesis, sleep loss prevents the normal overnight reduction in noradrenergic tone that usually occurs during rapid eye movement (REM) sleep 112 – 114 . The resulting hypernoradrenergic tone may thus lead to heightened and over-generalized responsivity within the affective salience network that is innervated by noradrenaline: the amygdala and the viscerosensory cortical regions 84 . Consequently, there may be a loss of specificity in affective signalling within this network, possibly helping to explain the SD-associated inaccuracy in emotional discrimination. This REM sleep model 84 offers several testable predictions regarding the reliable co-occurrence of sleep disruption (including REM sleep disruption) in clinical anxiety disorders ( BOX 3 ). However, noradrenaline alone is unlikely to be the sole neurochemical factor underlying the affective dysregulation caused by SD. Integrating changes in other sleep-dependent neurochemicals, such as dopamine, may provide a more accurate explanatory model.
Hippocampal memory processing
Many studies have characterized the beneficial impact of sleep on the post-learning, offline consolidation of hippocampus-dependent memories (for a review, see REF. 115 ). By contrast, few studies have explored the adverse effects of SD on this process 115 ; instead, the majority of such neuroimaging investigations have examined the impact of sleep loss on initial hippocampus-dependent memory encoding, on which we focus here.
In rodents, SD substantially decreases the ability to induce hippocampal long-term potentiation (LTP; an electrophysiological measure of neuroplasticity) and, if LTP is induced, the enhancement subsequently decays more rapidly 116 . Further, sleep loss reduces hippocampal synthesis of proteins associated with neuroplasticity and impairs hippocampal neurogenesis 117 .
The extracellular build-up of the metabolic product adenosine during extended wake affects plasticity. High levels of adenosine disrupt intracellular cAMP signalling in rodents and decreases hippocampal AMPA and NMDA receptor signalling; all of which are necessary for stable LTP 115 . Given that adenosine is cleared from the brain during sleep, these effects on plasticity may result from two mutually non-exclusive reasons: abnormally extended wake or a loss of sleep.
Building on these findings, early human neuroimaging and behavioural studies established that one night of SD impairs learning and encoding-related activity within the medial temporal lobe 118 , specifically the hippocampus 36 ( FIG. 4 ). Moreover, selective deprivation of non-rapid eye movement (NREM) slow-wave sleep using auditory stimulation, which preserves total sleep time, also lowers encoding-related activity in the hippocampus and associated learning 119 . Conversely, enhancing the power of NREM slow-wave activity, using transcranial stimulation, increases hippocampal learning ability after sleep 120 , further supporting a causal role for slow-wave sleep in hippocampal memory encoding.

Sleep deprivation (SD) decreases encoding-related activity within the hippocampus (light blue), compared with normal sleep-rested conditions. Moreover, in sleep-deprived individuals, hippocampal connectivity with encoding-relevant cortical regions of the intraparietal sulcus (IPS), posterior cingulate cortex (PCC) and primary visual cortex (V1) (purple regions) during encoding is lower than in sleep-rested individuals. By contrast, hippocampal encoding-related connectivity with subcortical arousal regions of the thalamus and brainstem (red) after SD is increased.
In addition, SD alters learning-related hippocampal connectivity. Functional coupling between the hippocampus and perceptual regions of the occipital (visual) cortex and nearby regions in the medial temporal lobe during visual episodic-memory encoding is decreased under SD conditions 36 ( FIG. 4 ). By contrast, SD is also associated with increases in hippocampal connectivity with subcortical arousal regions, including the brainstem and the thalamus 36 ( FIG. 4 ). The latter observation has been interpreted as reflecting a compensatory effort to mobilize basic arousal networks, albeit insufficient to rescue behavioural performance 36 .
A recent report has demonstrated that the structural morphology of the human hippocampus, and specifically the volume of the CA3–dentate gyrus subfield region, predicts the degree of vulnerability to the impact of SD on memory encoding 121 . Moreover, this same measure also predicted the amplitude of NREM slow-wave oscillations during recovery sleep and, by way of this mediating influence, determined how well memory-encoding ability was restored when assessed again after recovery sleep. Thus, human hippocampal subfield anatomy may represent a novel trait-like factor and potential biomarker of susceptibility of memory processing to, and recovery from, the disrupting effects of SD.
The hippocampus does not operate in isolation during memory encoding, however, but acts in a broad network of anatomically and functionally connected cortical regions. Some of these are proposed to participate in encoding memory representations themselves (for example, sensory perceptual regions), whereas others are involved in operations that support the act of encoding, such as the DLPFC and posterior parietal regions, which are required for directed attention 32 . Consistent with this, human neuroimaging studies have revealed that SD is associated with larger-network impairments that co-occur or are functionally linked with deficits in hippocampus-dependent encoding of new memories. For example, SD for 24 hours decreases activity in regions of the DLPFC and posterior parietal cortex required for goal-directed attention during a visual episodic- memory encoding task 122 . Reductions in the activity of the scene-selective fusiform cortex following SD are less clear to interpret and may reflect impairment in the functional role of the visual cortex in processing visual scenes and/or the failure of the top-down control of attention by frontoparietal networks 123 . Nevertheless, the size of the alterations in activity of all three of these cortical regions (frontal, parietal and occipital) predict the degree of memory impairment under SD conditions and the severity of attentional task lapses.
As with working-memory and selective attention tasks, significant differences in DMN activity within cortical regions have been reported during episodic-memory encoding following SD 124 . Indeed, the size of reductions and increases in the activity of the anterior cingulate and precuneus (which are both nodes of the DMN), respectively, discriminated sleep-deprived from sleep-rested participants with 93% sensitivity and 92% specificity 124 . Unstable control of the DMN might therefore be a common neural phenotype underlying deficits in various cognitive tasks (including attention, working memory and episodic memory) in the sleep-deprived human brain ( BOX 2 ).
Only one study to date has examined the under lying neurochemical basis of memory-encoding deficits associated with SD. Administration of an acetylcholinesterase inhibitor, which prolongs the central synaptic effects of acetylcholine, partially restored activity in frontoparietal and fusiform regions in sleep-deprived participants towards levels observed under sleep-rested conditions 122 ( FIG. 4 ). Interestingly, the greatest benefit of this acetylcholinesterase inhibitor treatment was observed in those individuals who, with SD, demonstrated the largest decreases in brain activity during a semantic judgement task and the worst performance in word recognition. That is, those most susceptible to sleep loss on these tasks were those who recovered most function after being treated with the drug.
Given that acetylcholinesterase inhibitors enhance attentional processes that support episodic memory 125 and directly affect sensory processing 126 , some part of this benefit might be mediated by cholinergic influences on arousal. An alternative or additional plausible hypothesis is that the actions of the inhibitor facilitate hippocampal plasticity mechanisms that consequentially increase hippocampal engagement of sensory and attentional networks in the cortex. Accordingly, acetylcholinesterase inhibitors enhance activation of the visual and parietal cortices in sleep-deprived individuals during a visual short-term-memory task and, compared with placebo, improve task performance 127 .
At a translational level, impaired hippocampus-dependent memory encoding following sleep loss is relevant to several disorders and conditions that involve deficiencies in both sleep and memory encoding. Two such examples are ageing and dementia. Older, cognitively normal adults show marked impairments in NREM sleep, and these deficits are magnified in size in Alzheimer disease 128 . Disruptions of NREM sleep and associated reductions in slow-wave activity and sleep spindles in older adults and, even more dramatically, in patients with Alzheimer disease predict reductions in encoding-related activity in the hippocampus on the following day, which, in turn, predict the degree of learning impairment 119 , 129 . Sleep disruption therefore may contribute to cognitive decline, and especially impairments in hippocampus-dependent memory processing, with ageing. This evidence raises the possibility that sleep restoration may be a novel therapeutic intervention, as well as a midlife preventative measure against age-related decline in hippocampus-dependent memory (for a more detailed discussion, see REF. 128 ).
Conclusions
Several insights emerge from this Review. First, SD triggers a complex set of bidirectional changes in brain activity and connectivity — depending on the specific functional operation and anatomical regions in question. Equally important, however, are moment-to-moment fluctuations in brain activity that occur during performance across the timescale of minutes, reflecting a neural phenotype of regional and network instability. This is especially apparent in the domains of attention and working memory. Second, the heterogeneous array of brain changes (averaged and moment-to-moment) results in an equally diverse set of disruptions in human behaviour across nearly all domains of cognition and affect. Nested within this statement is another related subtlety: not all changes in brain function that are associated with sleep loss are maladaptive and thus represent deficiencies. Instead, some aspects of these neural alterations seem to correlate with preserved task performance, indicating that they may be compensatory and thus adaptive. That the human brain can compensate for SD to a certain degree seems tenable, given that individuals use compensatory effort and behavioural strategies (for example, talking or standing) when attempting to stay awake when sleep deprived. However, the extent and duration of compensatory brain function, which networks and associated operations they are in, and which behaviours are maintained as a consequence, remain poorly characterized. For example, do the effects of SD depend on interactions among hierarchies of functional brain networks, such that deficits or compensation in a fundamental, base-level network (for example, the attention network) dictates respective impairment (or compensation) in higher-level, dependent operations (such as intentional memory encoding or conscious emotional processing)?
Equally important, this Review reveals our current inadequacies of knowledge regarding the sleep- deprived human brain. For example, we have remarkably little understanding of the whole-brain consequences of long-term, chronic sleep loss, as most studies have focused on acute, total SD ( BOX 1 ). Such knowledge would be crucial considering that chronic partial sleep restriction, from weeks to years, is representative of (and thus relevant to) the sleep deficiency observed in the developed world. We also lack any comprehensive understanding of whether and how human brain networks may recover from acute and chronic sleep restriction. Are the underlying neural mechanisms that support recovery from these different forms of sleep loss the same, partially overlapping, or entirely separable? Relatedly, does neural recovery occur across the same time period or over a different temporal duration following different forms and doses of sleep loss? What types of recovery sleep stages and/or aspects of sleep physiology transact functional brain restoration following sleep loss? These questions are important from a societal and public-health perspective, both in professional circumstances in which the privation of sleep is rife (for example, in the military, in aviation and in medicine) and in subtler forms (for example, the public trend of short sleep durations during the week followed by longer sleep durations at weekends).
Another key unresolved issue extends beyond the phenotypic between-participant differences in vulnerability to the neural and behavioural effects of SD within a cognitive domain (such as attention) ( BOX 1 ) and centres on emerging evidence suggesting within-participant, between-domain differences in vulnerability: an individual who is resilient to the effects of SD in one functional domain (such as attention) may conversely be vulnerable to that same dose of sleep loss in other functional domains (such as memory or emotion processing) 130 . This suggests that different brain networks in the same individual may be differentially susceptible to sleep loss. These hypothetical within-subject differences in neural network vulnerability and resilience to SD have not yet been experimentally tested.
Finally, the basic scientific findings regarding sleep loss have not yet been routinely applied in the clinic, despite the examples that we describe in BOX 3 . Sleep abnormalities are robustly observed in every major disorder of the brain, both neurological and psychiatric. Sleep disruption merits recognition as a key relevant factor in these disorders at all levels, from diagnosis and underlying aetiology, to therapy and prevention. More collaborative work between basic and clinical scientists in the field will be necessary to accomplish this goal. Notably, the answers to all these questions have perhaps never been more pressing considering the professional, societal and clinical implications that continue to scale in lockstep with the precipitous decline in sleep duration throughout industrialized nations.
Acknowledgments
This work was supported by awards R01-AG031164, R01-AG054019, RF1-AG054019 and R01-MH093537 to M.P.W. from the US National Institutes of Health.
Competing interests statement
The authors declare no competing interests.
The effect of total sleep deprivation on working memory: evidence from diffusion model
Affiliations.
- 1 Philosophy and Social Science Laboratory of Reading and Development in Children and Adolescents (South China Normal University), Ministry of Education, Guangzhou 510631, China.
- 2 Center for Sleep Research, Center for Studies of Psychological Application, Guangdong Key Laboratory of Mental Health and Cognitive Science, School of Psychology, South China Normal University, Guangzhou 510631, China.
- PMID: 38181126
- DOI: 10.1093/sleep/zsae006
Study objectives: Working memory is crucial in human daily life and is vulnerable to sleep loss. The current study investigated the impact of sleep deprivation on working memory from the information processing perspective, to explore whether sleep deprivation affects the working memory via impairing information manipulation.
Methods: Thirty-seven healthy adults attended two counterbalanced protocols: a normal sleep night and a total sleep deprivation (TSD). The N-back and the psychomotor vigilance task (PVT) assessed working memory and sustained attention. Response time distribution and drift-diffusion model analyses were applied to explore cognitive process alterations.
Results: TSD increased the loading effect of accuracy, but not the loading effect of response time in the N-back task. TSD reduced the speed of information accumulation, increased the variability of the speed of accumulation, and elevated the decision threshold only in 1-back task. Moreover, the slow responses of PVT and N-back were severely impaired after TSD, mainly due to increased information accumulation variability.
Conclusions: The present study provides a new perspective to investigate behavioral performance by using response time distribution and drift-diffusion models, revealing that sleep deprivation affected multicognitive processes underlying working memory, especially information accumulation processes.
Keywords: drift-diffusion models; sleep deprivation; state instability; sustained attention; working memory.
© The Author(s) 2024. Published by Oxford University Press on behalf of Sleep Research Society. All rights reserved. For permissions, please e-mail: [email protected].
- Attention / physiology
- Memory, Short-Term* / physiology
- Psychomotor Performance / physiology
- Reaction Time / physiology
- Sleep / physiology
- Sleep Deprivation* / complications
- Sleep Deprivation* / psychology
Grants and funding
- 2023A1515011873/Guangdong Basic and Applied Basic Research Foundation

How sleep deprivation and sleep apnea impact heart health
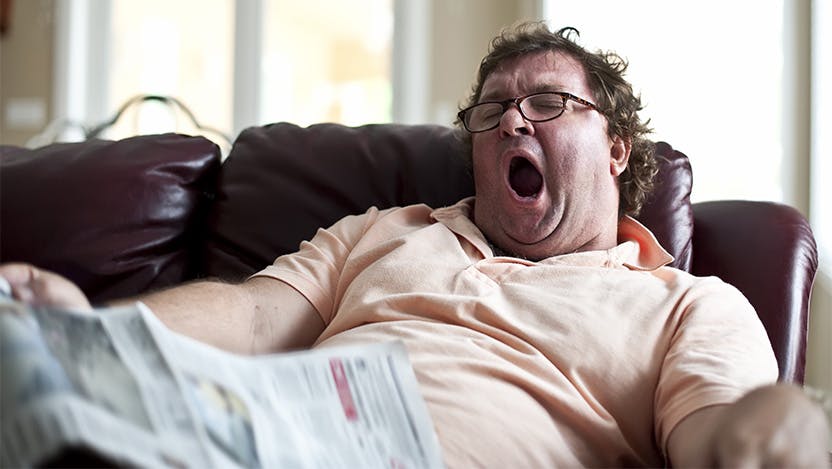
In today’s fast-paced world, we often forfeit sleep in the name of productivity.
Unfortunately, sacrificing shut-eye can have significant consequences when it comes to heart health. University of Chicago Medicine cardiologist Kathleen Drinan, DO, and sleep surgeon Phillip LoSavio, MD , weigh in on the dangers of sleep deprivation and why it’s important to address it before it impacts your life.
What happens when we are sleep-deprived?
Approximately one in three adults sleeps less than the recommended seven hours per night. This chronic sleep deprivation takes its toll on processes linked directly to cardiovascular function, leading to issues such as:
- Elevated blood pressure. Interrupted or inadequate sleep cycles can lead to higher-than-average blood pressure levels, which can strain the heart and blood vessels over time.
- Inflammation and stress. Chronic sleep deprivation can lead to higher levels of stress hormones like cortisol, which may contribute to the development of cardiovascular diseases over time.
- Metabolic changes and weight gain. Poor sleep patterns can affect appetite-regulating hormones like leptin and ghrelin. This imbalance can lead to overeating and weight gain, which are risk factors for heart disease and diabetes.
A connection between sleep and heart problems
Surprisingly, even a one-hour shift in sleep can have a measurable impact on heart health.
When clocks "spring forward" for daylight saving time, and we lose an hour of sleep, studies show an increase in the number of heart attacks.
“For about three days after we change our clocks forward, there is about a 24% increased risk of heart attacks,” Drinan said. And when clocks fall back, "we see a 21% reduction in heart attack risks as we gain an hour of sleep."
Studies also show that healthy adults who sleep five hours or less have a 200% to 300% higher risk of coronary artery calcification, the buildup of calcium in the heart’s main arteries.
“We think the lack of sleep increases heart disease risks by forcing our bodies to rely on the sympathetic nervous system, often referred to as the ‘fight or flight' nervous system,” Drinan said. “This leads to the release of more adrenaline and high cortisol levels, which leads to increased risks of heart disease.”
The dangers of undiagnosed sleep apnea
One of the most prevalent but underdiagnosed sleep disorders is sleep apnea , which is characterized by interrupted or obstructed breathing during sleep.
LoSavio estimates somewhere between 50 million to 60 million people in the United States have sleep apnea but only 6 million to 7 million have been diagnosed.
Common symptoms of sleep apnea include loud snoring, abrupt awakenings with shortness of breath, waking up with a dry mouth or sore throat, morning headaches, difficulty staying asleep, excessive daytime sleepiness, and irritability.
Left untreated, sleep apnea can lead to health issues such as high blood pressure, heart problems, type 2 diabetes, and daytime fatigue that can affect work or daily activities.
Drinan noted that these factors can create triggers for heart rhythm disorders, especially atrial fibrillation.
“I refer patients with atrial fibrillation and other arrhythmias for sleep evaluations at the UChicago Medicine Sleep Center . The presence of sleep disorders, especially untreated sleep apnea, can trigger many cardiac arrhythmias and elevate blood pressure, as well as lead to weight gain,” she said.
Treatment can involve lifestyle changes such as weight loss, sleeping position adjustments, using a CPAP (Continuous Positive Airway Pressure) machine, oral appliances, or in some cases, surgery to correct airway obstructions.
“The gap between the 6 million diagnosed and 60 million undiagnosed with sleep apnea is that people are afraid to seek out treatment,” LoSavio said.
“Nobody wants to get a sleep study because they picture being hooked up to a bunch of electrodes in a lab. The truth is about 90% of the sleep studies we do are home studies. We send most patients home with a wrist monitor to wear for one night. From that we can get highly accurate information about their sleep apnea.”
How can we get better sleep?
Rest assured most Americans need to overhaul their sleep hygiene, a set of practices and habits that promote good sleep quality and overall health. The first step is setting and sticking to a regular schedule of going to sleep and rising at the same time every day. Sleep regularity might even be more important than the length of sleep, according to LoSavio.
Other recommendations for better sleep hygiene include:
- Creating a comfortable sleep environment that is dark, cool, and quiet
- Avoiding stimulants like caffeine and nicotine before bedtime
- Regularly exercising to promote better sleep patterns
- Reducing screen time at least an hour before going to sleep
- Managing stress with mindfulness, journaling and therapy
- Limiting daytime naps
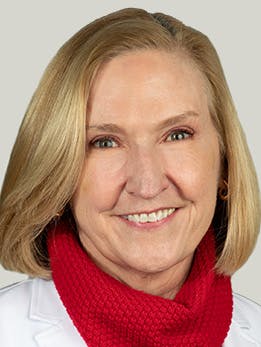
Kathleen Drinan, DO
Kathleen Drinan, DO, FACC, FACOI, is a highly skilled clinical cardiologist with over 30 years of cardiac experience. Dr. Drinan is trained in preventive and non-invasive treatments for a wide range of heart conditions, including understanding the difference between heart disease in men and women.
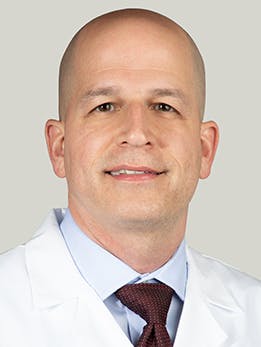
Phillip LoSavio, MD
Board-certified otolaryngologist, Phillip LoSavio, MD, MS, is a sleep surgeon who specializes in treating sleep apnea and related upper airway problems, such as nasal airway obstruction, deviated septum, tonsil enlargement, sinusitis and snoring.
Sleep Disorders in Adults
Our board-certified sleep medicine experts specialize in the assessment of respiratory and non-respiratory sleep disorders. Our services include sleep consultations and sleep studies.
Problem of Sleep Deprivation Cause and Effect Essay
Introduction.
- What is Sleep Deprivation?
Causes of Sleep Deprivation
Effects of sleep deprivation, managing sleep deprivation, works cited.
The functioning of the human body is influenced by a number of factors, which are mainly determined by the health status of an individual. Oftentimes, people seek medication when the body deviates from its normal and usual functioning mechanisms. Through different activities and processes, the body is able to use energy and replenish itself. Sleeping is one of the activities that has a direct effect on the functioning of the body.
This sleep deprivation essay explores how the functioning of the human body is influenced by various factors, primarily determined by an individual’s health status. While most people do not understand the implications of sleep, human effectiveness solely depends on the amount of time dedicated to sleeping. However, for various reasons, people fail to get enough sleep daily, weekly, or on a regular basis.
What Is Sleep Deprivation?
This cause and effect of sleep deprivation essay defines sleep deprivation as a condition occurring among human beings when they fail to get enough sleep. Sleep deprivation is defined as a condition that occurs when human beings fail to get enough sleep. Many experts argue that sleep deficiency is widespread even though most people do not consider it to be a serious issue, which affects their (Gaine et al.). Sleep deprivation has become a major problem in the United States, with almost 47 million suffering from the condition (Wang and Xiaomin). This lack of sleep can lead to a variety of physical and mental health issues, impacting daily functioning and quality of life.
The present essay about sleep deprivation defines sleep deprivation as a condition that occurs among human beings when they fail to get enough sleep. Many experts argue that sleep deficiency is widespread even though most people do not consider it to be a serious issue that affects their lives. Sleep deprivation has become a major problem in the United States, with almost forty-seven million suffering from the condition (Wang and Xiaomin). Among other reasons, one may get insufficient sleep in a day as a result of various factors. Some people sleep at the wrong time due to busy daily schedules, while others have sleep disorders, which affect their sleeping patterns. The following segment of the paper discusses the causes of deprivation.
Sleep deprivation may occur as a result of factors that are not known to the patients. This is based on the fact that sleep deprivation may go beyond the number of hours one spends in bed. In some cases, the quality of sleep matters in determining the level of deprivation.
In this context, it is possible for one to be in bed for more than eight hours but suffer from the negative effects of sleep deprivation. Whilst this is the case, there are people who wake every morning feeling tired despite having spent a recommended number of hours in bed (Griggs et al.14367).
Sleep deprivation can be caused by medical conditions, which may include but are not limited to asthma, arthritis, muscle cramps, allergies, and muscular pain. These conditions have been classified by researchers as common medical conditions that largely contribute to most of the cases of sleep deprivation being witnessed in the United States.
Similarly, these medical conditions have a direct impact on not only the quality but also the time one takes in bed sleeping. It is worth noting that sometimes people are usually unconscious to realize that their sleep is not deep enough (Wang and Xiaomin). This also explains the reason why it is not easy for a person to recall any moment in life when he or she moved closer to waking up.
Treatment of cases like sleep apnea is important because it affects the quality of sleep without necessarily awakening the victim. This is because medical surveys have revealed fatal effects of sleep apnea, especially on the cardiovascular system. Besides these, one is likely to experience breathing difficulties caused by insufficient oxygen.
Even though the treatment of sleep deprivation is important, it has been found that some drugs used to treat patients may worsen the case or lead to poor quality of sleep. It is, therefore, necessary for the doctor to determine the best drugs to use. Discussions between doctors and victims are imperative in order to understand patients’ responses (Conroy et al. 185).
Sleep deprivation is also caused by sleep cycle disruptions, which interfere with the fourth stage of sleep. Oftentimes, these disruptions are described as night terrors, sleepwalking, and nightmares.
Though these disorders are known not to awaken a person completely, it is vital to note that they may disrupt the order of sleep cycles, forcing a person to move from the fourth stage to the first one. Victims of these disruptions require attention in order to take corrective measures.
In addition, there are known environmental factors which contribute to several cases of sleep deprivation. However, doctors argue that the impact on the environment is sometimes too minimal to be recognized by people who are affected by sleep deficiency (Gaine et al.). In other words, these factors affect the quality of sleep without necessarily arousing a person from sleep.
Common examples include extreme weather conditions, like high temperatures, noise, and poor quality of the mattress. As a result, they may contribute to a person’s awakening, depending on the intensity when one is sleeping.
Moreover, the impact of these factors may develop with time, thus affecting one’s quality of sleep. In addition, most of the environmental factors that contribute to sleep deprivation can be fixed easily without medical or professional skills. Nevertheless, the challenge is usually how to become aware of their existence.
Lastly, sleep deprivation is caused by stress and depression, which have been linked to other health disorders and complications. Together with some lifestyles in America, these factors are heavily contributing to sleep deficiency in most parts of the world. Even though they might not be acute enough to awaken an individual, their cumulative effects usually become significant.
There are countless stressors in the world that affect youths and adults. While young people could be concerned with passing exams, adults are normally preoccupied with pressure to attain certain goals in life. These conditions create a disturbed mind, which may affect a person’s ability to enjoy quality sleep.
Sleep deprivation has a host of negative effects which affect people of all ages. The commonest effect is stress. Most people who suffer from sleep deficiency are likely to experience depression frequently as compared to their counterparts who enjoy quality sleep (Conroy et al. 188). As a result, stress may lead to poor performance among students at school.
Research has revealed that students who spend very few hours in bed or experience disruptions during sleep are likely to register poor performance in their class assignments and final exams. Additionally, sleep deprivation causes inefficiency among employees.
For instance, drivers who experience this disorder are more likely to cause accidents as compared to those who are free from it (Griggs et al.14367). This is based on the fact that un-refreshed people have poor concentration and low mastery of their skills.
Besides stress and anxiety, sleep deprivation has a wide-range of health-related effects. For instance, medical experts argue that people who spend less than six hours in bed are likely to suffer from high blood pressure. Quality sleep gives the body an opportunity to rest by slowing down the rate at which it pumps blood to the rest of the body (Wang and Xiaomin).
Inadequate sleep implies that the heart has to work without its normal and recommended rest. Additionally, sleep deprivation is known to affect the immune system. People who experience this disorder end up with a weakened immune system, leaving the body prone to most illnesses. This reduced immune response accumulates and may become fatal with time.
Sleep paralysis is also a common effect of inadequate sleep. This is due to disruption of the sleep cycle. It primarily occurs when the body is aroused during the fourth stage of the sleep cycle. In this case, the body is left immobile as the mind regains consciousness. Due to this conflict, one may experience pain and hallucinations.
Based on the negative effects of sleep deprivation, there is a need to manage this disorder among Americans. Firstly, it is necessary for people to seek medical advice concerning certain factors which could be contributing to this condition, like stress and infections (Wang and Xiaomin).
Proper counseling is also vital in stabilizing a person’s mental capacity. Physical exercises are also known to relieve a person from stressful conditions, contributing to sleep deficiency. Lastly, it is essential to ensure that the environment is free from noise and has regulated weather conditions.
Sleep deprivation remains a major problem in America, affecting millions of people. As discussed above, sleep deprivation is caused by a host of factors, ranging from environmental to health-related issues. Moreover, sleep deficiency has countless effects, most of which may become fatal in cases where the disorder is chronic.
Conroy, Deirdre A., et al. “ The Effects of COVID-19 Stay-at-home Order on Sleep, Health, and Working Patterns: A Survey Study of US Health Care Workers. ” Journal of Clinical Sleep Medicine , vol. 17, no. 2, Feb. 2021, pp. 185–91.
Gaine, Marie E., et al. “ Altered Hippocampal Transcriptome Dynamics Following Sleep Deprivation. ” Molecular Brain, vol. 14, no. 1, Aug. 2021.
Griggs, Stephanie, et al. “ Socioeconomic Deprivation, Sleep Duration, and Mental Health During the First Year of the COVID-19 Pandemic. ” International Journal of Environmental Research and Public Health, vol. 19, no. 21, Nov. 2022, p. 14367.
Wang, Jun, and Xiaomin Ren. “ Association Between Sleep Duration and Sleep Disorder Data From the National Health and Nutrition Examination Survey and Stroke Among Adults in the United States .” Medical Science Monitor , vol. 28, June 2022.
- Chicago (A-D)
- Chicago (N-B)
IvyPanda. (2019, June 2). Problem of Sleep Deprivation. https://ivypanda.com/essays/sleep-deprivation/
"Problem of Sleep Deprivation." IvyPanda , 2 June 2019, ivypanda.com/essays/sleep-deprivation/.
IvyPanda . (2019) 'Problem of Sleep Deprivation'. 2 June.
IvyPanda . 2019. "Problem of Sleep Deprivation." June 2, 2019. https://ivypanda.com/essays/sleep-deprivation/.
1. IvyPanda . "Problem of Sleep Deprivation." June 2, 2019. https://ivypanda.com/essays/sleep-deprivation/.
Bibliography
IvyPanda . "Problem of Sleep Deprivation." June 2, 2019. https://ivypanda.com/essays/sleep-deprivation/.
- The Issue of Chronic Sleep Deprivation
- Sleep Deprivation: Biopsychology and Health Psychology
- Sleep Deprivation: Research Methods
- How the Brain Learns: Neuro-Scientific Research and Recent Discoveries
- Parkinson's Disease Treatment Approaches
- Concepts of Batten Disease
- Dyslexia: Definition, Causes, Characteristics
- How Dysphagia Disorder (Swallowing Disorder) is Caused in Parkinson’s Disease

Potential power and pitfalls of harnessing artificial intelligence for sleep medicine
Ai has potential uses in the sleep field for clinical applications, lifestyle management, and population health.
In a new research commentary, the Artificial Intelligence in Sleep Medicine Committee of the American Academy of Sleep Medicine highlights how artificial intelligence stands on the threshold of making monumental contributions to the field of sleep medicine. Through a strategic analysis, the committee examined advancements in AI within sleep medicine and spotlighted its potential in revolutionizing care in three critical areas: clinical applications, lifestyle management, and population health. The committee also reviewed barriers and challenges associated with using AI-enabled technologies.
"AI is disrupting all areas of medicine, and the future of sleep medicine is poised at a transformational crossroad," said lead author Dr. Anuja Bandyopadhyay, chair of the Artificial Intelligence in Sleep Medicine Committee. "This commentary outlines the powerful potential and challenges for sleep medicine physicians to be aware of as they begin leveraging AI to deliver precise, personalized patient care and enhance preventive health strategies on a larger scale while ensuring its ethical deployment."
According to the authors, AI has potential uses in the sleep field in three key areas:
- Clinical Applications :In the clinical realm, AI-driven technologies offer comprehensive data analysis, nuanced pattern recognition and automation in diagnosis, all while addressing chronic problems like sleep-related breathing disorders. Despite understated beginnings, the utilization of AI can offer improvements in efficiency and patient access, which can contribute to a reduction in burnout among health care professionals.
- Lifestyle Management :Incorporating AI also offers clear benefits for lifestyle management through the use of consumer sleep technology. These devices come in various forms like fitness wristbands, smartphone apps, and smart rings, and they contribute to better sleep health through tracking, assessment and enhancement. Wearable sleep technology and data-driven lifestyle recommendations can empower patients to take an active role in managing their health, as shown in a recent AASM survey, which reported that 68% of adults who have used a sleep tracker said they have changed their behavior based on what they have learned. But, as these AI-driven applications grow ever more intuitive, the importance of ongoing dialogue between patients and clinicians about the potential and limitations of these innovations remains vital.
- Population Health : Beyond individual care, AI technology reveals a new approach to public health regarding sleep. "AI has the exciting potential to synthesize environmental, behavioral and physiological data, contributing to informed population-level interventions and bridging existing health care gaps," noted Bandyopadhyay.
The paper also offers warnings about the integration of AI into sleep medicine. Issues of data privacy, security, accuracy, and the potential for reinforcing existing biases present new challenges for health care professionals. Additionally, reliance on AI without sufficient clinical judgment could lead to complexities in patient treatment.
"While AI can significantly strengthen the evaluation and management of sleep disorders, it is intended to complement, not replace, the expertise of a sleep medicine professional," Bandyopadhyay stated.
Navigating this emerging landscape requires comprehensive validation and standardization protocols to responsibly and ethically implement AI technologies in health care. It's critical that AI tools are validated against varied datasets to ensure their reliability and accuracy in all patient populations.
"Our commentary provides not just a vision, but a roadmap for leveraging the technology to promote better sleep health outcomes," Bandyopadhyay said. "It lays the foundation for future discussions on the ethical deployment of AI, the importance of clinician education, and the harmonization of this new technology with existing practices to optimize patient care."
- Sleep Disorders
- Obstructive Sleep Apnea
- Mental Health
- Information Technology
- Artificial Intelligence
- Circadian rhythm sleep disorder
- Sleep deprivation
- Sleep disorder
- Night terror
- Delayed sleep phase syndrome
- Rapid eye movement
- Narcolepsy (sleep disorder)
Story Source:
Materials provided by American Academy of Sleep Medicine . Note: Content may be edited for style and length.
Journal Reference :
- Anuja Bandyopadhyay, Margarita Oks, Haoqi Sun, Bharati Prasad, Sam Rusk, Felicia Jefferson, Roneil Gopal Malkani, Shahab Haghayegh, Ramesh Sachdeva, Dennis Hwang, Jon Agustsson, Emmanuel Mignot, Michael Summers, Daniel Fabbri, Maryann Deak, Matthew Anastasi, Andrew Sampson, Steve Van Hout, Azizi Seixas. Strengths, weaknesses, opportunities and threats of using AI-enabled technology in sleep medicine: a commentary . Journal of Clinical Sleep Medicine , 2024; DOI: 10.5664/jcsm.11132
Cite This Page :
Explore More
- Nature's 3D Printer: Bristle Worms
- Giant ' Cotton Candy' Planet
- A Young Whale's Journey
- No Inner Voice Linked to Poorer Verbal Memory
- Bird Flu A(H5N1) Transmitted from Cow to Human
- Universe's Oldest Stars in Our Galactic Backyard
- Polygenic Embryo Screening for IVF: Opinions
- VR With Cinematoghraphics More Engaging
- 2023 Was the Hottest Summer in 2000 Years
- Fastest Rate of CO2 Rise Over Last 50,000 Years
Trending Topics
Strange & offbeat.

IMAGES
VIDEO
COMMENTS
Differences in age and sex were not discussed in all but two studies reported in one paper (Honn et al., 2020), where no significant group differences in age or sex were found (p = 0.24 and 0.26 ... Less effective executive functioning after one night's sleep deprivation. Journal of Sleep Research, 14 (1), 1-6. 10.1111/j.1365-2869.2005. ...
INTRODUCTION. Sleep is vital for health and well-being in children, adolescents, and adults. 1-3 Healthy sleep is important for cognitive functioning, mood, mental health, and cardiovascular, cerebrovascular, and metabolic health. 4 Adequate quantity and quality of sleep also play a role in reducing the risk of accidents and injuries caused by sleepiness and fatigue, including workplace ...
Total sleep deprivation (TSD) may induce fatigue, neurocognitive slowing and mood changes, which are partly compensated by stress regulating brain systems, resulting in altered dopamine and cortisol levels in order to stay awake if needed. These systems, however, have never been studied in concert. At baseline, after a regular night of sleep, and the next morning after TSD, 12 healthy subjects ...
Research suggests that sleep deprivation both before and after encoding has a detrimental effect on memory for newly learned material. However, there is as yet no quantitative analyses of the size of these effects. We conducted two meta-analyses of studies published between 1970 and 2020 that investigated effects of total, acute sleep deprivation on memory (i.e., at least one full night of ...
This study aimed to determine whether a night of sleep. deprivation, equivalent to an "all-nighter", would ha ve a. negative impact on the motor and cognitive perf ormance of. students ...
To quantitatively describe the effects of sleep loss, we used meta-analysis, a technique relatively new to the sleep research field, to mathematically summarize data from 19 original research studies. Results of our analysis of 143 study coefficients and a total sample size of 1,932 suggest that overall sleep deprivation strongly impairs human ...
The need to quantify the necessary sleep time to keep our body functioning properly is the subject of numerous studies and represents more than simply "hours of sleep" for adequate physical and mental functioning, there is an important political and economic impact directly and indirectly caused by sleep deprivation (Hafner et al., 2017).
Sleep loss may lead to negative bias during social interaction. In the current study, we conducted a revised social evaluation task experiment to investigate how sleep deprivation influences the self-referential and cognitive processes of social feedback. The experiment consisted of a first impression task and a social feedback task. Seventy-eight participants completed the first impression ...
Sleep disruption is a common but poorly understood feature of neurodevelopmental disorders including autism spectrum disorder. A study by Bian et al. reveals that sleep disruption in adolescent ...
Sleep changes significantly throughout the human lifespan. Physiological modifications in sleep regulation, in common with many mammals (especially in the circadian rhythms), predispose adolescents to sleep loss until early adulthood. Adolescents are one-sixth of all human beings and are at high risk for mental diseases (particularly mood disorders) and self-injury. This has been attributed to ...
found that "up to 60% of all college students suffer from a poor sleep quality" (Schlarb et al., 2017, p. 1). Overtime, a lack of sleep can negatively affect a person's physiological health, psychological health, and cognitive function.
Decreases in functional connectivity between top-down control regions of the mPFC and the amygdala have been reported following a night of total SD or 5 nights of partial sleep restriction (4 hours sleep per night), as well as in participants reporting less than 6 hours of habitual sleep 81 - 83, 85, 86 ( FIG. 3 ).
papers were dropped, and two papers were added from hand- ... research related to sleep deprivation among workers was . ... An outline of the outcomes .
The current study investigated the impact of sleep deprivation on working memory from the information processing perspective, to explore whether sleep deprivation affects the working memory via impairing information manipulation. Methods: Thirty-seven healthy adults attended two counterbalanced protocols: a normal sleep night and a total sleep ...
below of sleep and students who spent 6 hours of sleep. 3.1 There is no significant difference in the average hours of sleep of students who is 18 to 20 years old and. 21 years old and above. 3.2 ...
Sleep deprivation can have a multitude of adverse effects on college students such as decreased attention spans, fluctuation in emotions, and memory consolidation. This study investigates the effect of sleep deprivation on the academic performance of college students in North Texas. Surveys including questions concerning one's GPA, average ...
The Effects Of Sleep Deprivation Among Nurses Jennifer M. Sison Urdaneta City University San Vicente West Urdaneta City Pangasinan [email protected] Abstract: This study assessed the effects of sleep deprivation encountered among nurses. It dealt with the profile of the respondents in
Left untreated, sleep apnea can lead to health issues such as high blood pressure, heart problems, type 2 diabetes, and daytime fatigue that can affect work or daily activities. Drinan noted that these factors can create triggers for heart rhythm disorders, especially atrial fibrillation. "I refer patients with atrial fibrillation and other ...
Effects of Sleep Deprivation. Sleep deprivation has a host of negative effects which affect people of all ages. The commonest effect is stress. Most people who suffer from sleep deficiency are likely to experience depression frequently as compared to their counterparts who enjoy quality sleep (Conroy et al. 188).
Objective: The purpose of this pilot study was to understand nursing students' perception of the relationship between sleep. deprivation and learning, and personal and patient safety when in a ...
AI has potential uses in the sleep field for clinical applications, lifestyle management, and population health. A new article highlights how artificial intelligence stands on the threshold of ...
DOI: 10.1016/j.sleep.2023.11.155 Corpus ID: 267662869; The effect of sleep deprivation on food choice certainty: a pilot study @article{Bazzani2024TheEO, title={The effect of sleep deprivation on food choice certainty: a pilot study}, author={Andrea Bazzani and P. Frumento and P. Nguyen and S. Ravaioli and G. Turchetti and U. Faraguna}, journal={Sleep Medicine}, year={2024}, url={https://api ...
Coronavirus disease-19 (COVID-19) has disproportionately affected certain demographics in England, exacerbating existing health disparities. Effective therapeutics are a critical line of defence against COVID-19, particularly for patients at elevated risk for severe disease. Surveillance systems were established to monitor the usage of COVID-19 therapeutics in hospital and community settings ...
The VFMF threshold was performed during sleep deprivation and 4 weeks of recovery phase. 1) The effect of sleep deprivation on mechanical pain threshold. In comparison between SD and NSD-C group, the significant difference appeared after 1 day of sleep deprivation and lasted 4 days. 2) The effect of sleep deprivation on FOS reactivity.