Better synonyms for enriching biomedical search
Affiliation.
- 1 National Center for Biotechnology Information, National Library of Medicine, National Institutes of Health, Bethesda, Maryland, USA.
- PMID: 33083825
- PMCID: PMC7727334
- DOI: 10.1093/jamia/ocaa151
Objective: In a biomedical literature search, the link between a query and a document is often not established, because they use different terms to refer to the same concept. Distributional word embeddings are frequently used for detecting related words by computing the cosine similarity between them. However, previous research has not established either the best embedding methods for detecting synonyms among related word pairs or how effective such methods may be.
Materials and methods: In this study, we first create the BioSearchSyn set, a manually annotated set of synonyms, to assess and compare 3 widely used word-embedding methods (word2vec, fastText, and GloVe) in their ability to detect synonyms among related pairs of words. We demonstrate the shortcomings of the cosine similarity score between word embeddings for this task: the same scores have very different meanings for the different methods. To address the problem, we propose utilizing pool adjacent violators (PAV), an isotonic regression algorithm, to transform a cosine similarity into a probability of 2 words being synonyms.
Results: Experimental results using the BioSearchSyn set as a gold standard reveal which embedding methods have the best performance in identifying synonym pairs. The BioSearchSyn set also allows converting cosine similarity scores into probabilities, which provides a uniform interpretation of the synonymy score over different methods.
Conclusions: We introduced the BioSearchSyn corpus of 1000 term pairs, which allowed us to identify the best embedding method for detecting synonymy for biomedical search. Using the proposed method, we created PubTermVariants2.0: a large, automatically extracted set of synonym pairs that have augmented PubMed searches since the spring of 2019.
Published by Oxford University Press on behalf of the American Medical Informatics Association 2020. This work is written by a US Government employee and is in the public domain in the US.

Publication types
- Research Support, N.I.H., Intramural
- Biomedical Research*
- Information Storage and Retrieval / methods*
- Linguistics*
- Probability
- Terminology as Topic
Office: 919.785.1304 | Fax: 919.785.1306
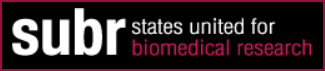
- Donate to SUBR
- Copyright and Privacy Statements
- Why Are Animals Needed in Research?
- What About Using Fewer Animals or No Animals?
- Product and Environmental Safety Research
- What is Biomedical Research?
- Biomedical Research Definitions
- Research Options
- How Animals Help
- Member Organizations
WHAT IS BIOMEDICAL RESEARCH?
Biomedical research is the broad area of science that looks for ways to prevent and treat diseases that cause illness and death in people and in animals. This general field of research includes many areas of both the life and physical sciences.
Utilizing biotechnology techniques, biomedical researchers study biological processes and diseases with the ultimate goal of developing effective treatments and cures. Biomedical research is an evolutionary process requiring careful experimentation by many scientists, including biologists and chemists. Discovery of new medicines and therapies requires careful scientific experimentation, development, and evaluation.
Why are Animals Used in Biomedical Research?
The use of animals in some types of research is essential to the development of new and more effective methods for diagnosing and treating diseases that affect both humans and animals. Scientists use animals to learn more about health problems, and to assure the safety of new medical treatments. Medical researchers need to understand health problems before they can develop ways to treat them. Some diseases and health problems involve processes that can only be studied in living organisms. Animals are necessary to medical research because it is impractical or unethical to use humans.
Animals make good research subjects for a variety of reasons. Animals are biologically similar to humans. They are susceptible to many of the same health problems, and they have short life-cycles so they can easily be studied throughout their whole life-span or across several generations. In addition, scientists can easily control the environment around animals (diet, temperature, lighting), which would be difficult to do with people. Finally, a primary reason why animals are used is that most people feel it would be wrong to deliberately expose human beings to health risks in order to observe the course of a disease.
Animals are used in research to develop drugs and medical procedures to treat diseases. Scientists may discover such drugs and procedures using alternative research methods that do not involve animals. If the new therapy seems promising, it is tested in animals to see whether it seems to be safe and effective. If the results of the animal studies are good, then human volunteers are asked to participate in a clinical trial. The animal studies are conducted first to give medical researchers a better idea of what benefits and complications they are likely to see in humans.
A variety of animals provide very useful models for the study of diseases afflicting both animals and humans. However, approximately 95 percent of research animals in the United States are rats, mice, and other rodents bred specifically for laboratory research. Dogs, cats, and primates account for less than one percent of all the animals used in research.
Those working in the field of biomedical research have a duty to conduct research in a manner that is humane, appropriate, and judicious. CBRA supports adherence to standards of care developed by scientific and professional organizations, and compliance with governmental regulations for the use of animals in research.
Scientists continue to look for ways to reduce the numbers of animals needed to obtain valid results, refine experimental techniques, and replace animals with other research methods whenever feasible.
© California Biomedical Research Association
- Skip to primary navigation
- Skip to main content
- Skip to footer
Human Research Protection Program
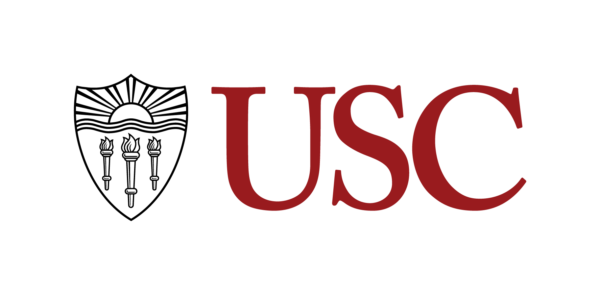
Biomedical Research
What is biomedical research.
Biomedical scientists study human physiology and the treatment or understanding of disease. Biomedical research applies the principles of the physical sciences to medicine. Most biomedical research is conducted by physicians or biomedical scientists, but many studies are conducted by biologists, chemists, physicists, and other medical and scientific professionals.
Most biomedical research involves clinical trials, which are phased studies using human volunteers, designed to answer safety and efficacy questions about biologics, devices, pharmaceuticals, new therapies or new ways of using known treatments. Trials are often conducted in small group initially but expanding in later stages once safety and efficacy are demonstrated. Most clinical trials are FDA regulated, but there are some exceptions.
Types and Methods
- research on therapies ( e.g. , drugs, exercise, surgical interventions, or medical devices)
- diagnostic procedures ( e.g. , CAT scans, prenatal diagnosis through amniocentesis)
- preventive measures ( e.g. , vaccines, diet, or fluoridated toothpaste)
- studies of the human body while exercising, fasting, feeding, sleeping, or learning
- responding to such things as stress or sensory stimulation
- Studies comparing the functioning of a particular physiological system at different stages of development ( e.g. , infancy, childhood, adolescence, adulthood, or old age)
- Studies defining normal childhood development so that deviations from normal can be identified
- Records research – often used to develop and refine hypotheses
- research on the biochemical changes associated with AIDS
- research on the neurological changes associated with senile dementia
- Research on the human genome and genetic markers – for the purpose of creating new avenues for understanding disease processes and their eventual control
- research with animals
- research on preexisting samples of materials (tissue, blood, or urine) collected for other purposes, where the information is recorded by the investigator in such a manner that subjects cannot be identified, directly or through identifiers linked to the subjects
- research based on records, when the data are recorded in such a manner that the individuals to whom the records pertain cannot be identified, either directly or through identifiers linked to them
Risk is the probability of harm or injury (physical, psychological, social, or economic) occurring as the result of participation in a research study. Biomedical researchers must consider the following risks when conducting their study:
- Social, psychological, or economic harm (See Social Behavioral Research for details)
- exercise-induced or repetition-exacerbated physical harm, such as carpal tunnel syndrome, stress fractures, asthma attacks, or heart attacks
- exposure to minor pain, discomfort (e.g. dizziness), or injury from invasive medical procedures
- possible side effects of drugs
Although most of the adverse effects that result from medical procedures or drugs are temporary, investigators must be aware of the potential for harm. The IRB will want to know how such outcomes will be minimized or addressed and is responsible for conducting a risk/benefit assessment.
When submitting an application in iStar you will see the following in section 1.5.
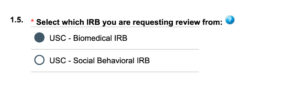
Genomic Data Sharing (GDS)/Stem Cell Research
- USC Stem Cell Regenerative Medicine Initiative
- USC Stem Cell Research Oversight Committee (SCRO)
- NIH Genomic Data Sharing Policy (formerly ‘GWAS’)
- California Institute for Regenerative Medicine (CIRM)
- Genetics and Public Policy Center
- NIH: Stem Cell Research
- U.K. Human Fertilisation and Embryological Authority
- The Hinxton Group: An International Consortium on Stem Cells, Ethics and Law
- The International Society for Stem Cell Research (ISSCR)
- Human Genome Project: Educational Kit
3720 S. Flower Street, Suite 325 Los Angeles, CA 90089
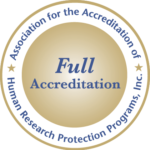
- Announcements
- Getting Started
- Internal Staff Webpage
- Education & Certification
- HRPP Performance & Metrics
- Policies and Procedures
- Post Approval Monitoring (PAM)
- Youtube Channel
- Biospecimen & Data Repositories
- Investigational Drugs and Devices
- Investigator-Initiated Trials
- Emergency Research
- SBIRB Social Behavioral Research
- Student Researchers
- Requesting USC to Rely on an External IRB
- Requesting USC IRB to Act as the sIRB
- Starting a Research Trial: the Basics
- Forms and Templates
- FWA and IRB Registration Numbers
- IRB Member Toolbox
- IRB Review: How to
- IRB Submission Guidelines
- Levels of IRB Review
- Not Human Subjects Research (NHSR)
- Post IRB Review and Approval
- Privacy, Confidentiality, and Anonymity in Human Subjects Research
- Urgent Review

- Cambridge Dictionary +Plus
Meaning of biomedical in English
Your browser doesn't support HTML5 audio
- The institute conducts research on the biomedical and social aspects of aging .
- They will research the issue of how to measure and alleviate pain and distress suffered by mice and rats used in biomedical experiments .
- Magnetic resonance imaging ( MRI ) is a non-invasive biomedical imaging technique .
- He is a former international cyclist with a degree in muscular physiology and biomedical engineering .
- anatomically correct
- anti-Darwinian
- entomological
- entomologist
- primitively
- recolonization
biomedical | Business English
Examples of biomedical, translations of biomedical.
Get a quick, free translation!
Word of the Day
sung by a group of people without any musical instruments

Shoots, blooms and blossom: talking about plants

Learn more with +Plus
- Recent and Recommended {{#preferredDictionaries}} {{name}} {{/preferredDictionaries}}
- Definitions Clear explanations of natural written and spoken English English Learner’s Dictionary Essential British English Essential American English
- Grammar and thesaurus Usage explanations of natural written and spoken English Grammar Thesaurus
- Pronunciation British and American pronunciations with audio English Pronunciation
- English–Chinese (Simplified) Chinese (Simplified)–English
- English–Chinese (Traditional) Chinese (Traditional)–English
- English–Dutch Dutch–English
- English–French French–English
- English–German German–English
- English–Indonesian Indonesian–English
- English–Italian Italian–English
- English–Japanese Japanese–English
- English–Norwegian Norwegian–English
- English–Polish Polish–English
- English–Portuguese Portuguese–English
- English–Spanish Spanish–English
- English–Swedish Swedish–English
- Dictionary +Plus Word Lists
- English Adjective
- Business Adjective
- Translations
- All translations
Add biomedical to one of your lists below, or create a new one.
{{message}}
Something went wrong.
There was a problem sending your report.
Related Words and Phrases
Bottom_desktop desktop:[300x250].
- ABBREVIATIONS
- BIOGRAPHIES
- CALCULATORS
- CONVERSIONS
- DEFINITIONS
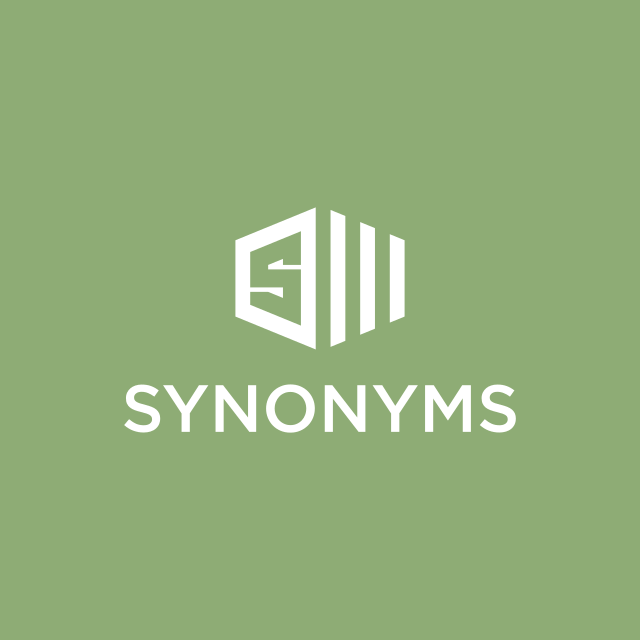
Vocabulary
What is another word for biomedical ?
Synonyms for biomedical bio·med·i·cal, this thesaurus page includes all potential synonyms, words with the same meaning and similar terms for the word biomedical ., princeton's wordnet.
biomedical adjective
relating to the activities and applications of science to clinical medicine
"biomedical research laboratory"
PPDB, the paraphrase database Rate these paraphrases: 0.0 / 0 votes
List of paraphrases for "biomedical":
bio-medical , biomedicine
How to pronounce biomedical?
How to say biomedical in sign language, words popularity by usage frequency, how to use biomedical in a sentence.
Gib Brogan :
They provide shelter and cover and they are the subject of an emerging field of biomedical research.
Jason Crawford :
If you can harness the abilities that marine animals have to make light, you can generate molecular systems for imaging in the lab or in medicine. Imaging is an incredibly important biomedical objective that these types of systems could help to propel into the future, sharks are wonderful animals that have been around for over 400 million years. Sharks continually fascinate humans, and they hold so many mysteries and superpowers.
Claire Doan :
Since the 1930s, fetal tissue has been a critical component of biomedical science and breakthroughs that fundamentally changed the practice of medicine.
Sanford Simon :
Kasia’s strategy is one that may help to get over the hump that has been classically bedeviling biomedical people in the U.S. to move something from the bench to the field without quite so many hurdles, the real-world [vaccine] application of this could potentially really have an impact, then I start daydreaming about other possibilities and if you can somehow add to the treatment of cancer or any other disease, adapting your technology in immunotherapies.
John Rogers :
We are excited about the fact that these simple ideas and schemes provide immediate paths to broad and previously inaccessible classes of 3D micro- and nano-structures in a way that is compatible with the highest-performance materials and processing techniques available, we feel that the findings have potential relevance to a wide range of microsystems technologies biomedical devices, optoelectronics, photovoltaics, 3D circuits, sensors and so on.
Use the citation below to add these synonyms to your bibliography:
Style: MLA Chicago APA
"biomedical." Synonyms.com. STANDS4 LLC, 2024. Web. 9 Apr. 2024. < https://www.synonyms.com/synonym/biomedical >.

Discuss these biomedical synonyms with the community:
Report Comment
We're doing our best to make sure our content is useful, accurate and safe. If by any chance you spot an inappropriate comment while navigating through our website please use this form to let us know, and we'll take care of it shortly.
You need to be logged in to favorite .
Create a new account.
Your name: * Required
Your email address: * Required
Pick a user name: * Required
Username: * Required
Password: * Required
Forgot your password? Retrieve it
Are we missing a good synonym for biomedical ?
Image credit, the web's largest resource for, synonyms & antonyms, a member of the stands4 network, free, no signup required :, add to chrome, add to firefox, browse synonyms.com, are you a human thesaurus, which of the following terms is not a synonym for "tolerable", nearby & related entries:.
- biology lab noun
- biology laboratory noun
- biomass noun
- biomass-based
- biomaterials
- biomedical adj
- biomedical cloning noun
- biomedical science noun
- biomedicine noun
- biometric authentication noun
Alternative searches for biomedical :
- Search for biomedical on Amazon


An official website of the United States government
The .gov means it’s official. Federal government websites often end in .gov or .mil. Before sharing sensitive information, make sure you’re on a federal government site.
The site is secure. The https:// ensures that you are connecting to the official website and that any information you provide is encrypted and transmitted securely.
- Publications
- Account settings
Preview improvements coming to the PMC website in October 2024. Learn More or Try it out now .
- Advanced Search
- Journal List
- Animals (Basel)
- PMC10093480

The Importance of Animal Models in Biomedical Research: Current Insights and Applications
Adriana domínguez-oliva.
1 Master’s Program in Agricultural and Livestock Sciences [Maestría en Ciencias Agropecuarias], Xochimilco Campus, Universidad Autónoma Metropolitana (UAM), Mexico City 04960, Mexico
Ismael Hernández-Ávalos
2 Clinical Pharmacology and Veterinary Anesthesia, Facultad de Estudios Superiores Cuautitlán, Universidad Nacional Autónoma de México (UNAM), Cuautitlán 54714, Mexico
Julio Martínez-Burnes
3 Facultad de Medicina Veterinaria y Zootecnia, Universidad Autónoma de Tamaulipas, Victoria City 87000, Mexico
Adriana Olmos-Hernández
4 Division of Biotechnology—Bioterio and Experimental Surgery, Instituto Nacional de Rehabilitación-Luis, Guillermo Ibarra Ibarra (INR-LGII), Mexico City 14389, Mexico
Antonio Verduzco-Mendoza
Daniel mota-rojas.
5 Neurophysiology, Behavior and Animal Welfare Assessment, DPAA, Universidad Autónoma Metropolitana (UAM), Mexico City 04960, Mexico
Associated Data
Not applicable.
Simple Summary
The present review highlights and examines the importance of animal models in relevant topics concerning current human and animal health. Over the past five years, different animal species have been used to study pandemics, such as the 2019 Coronavirus, diabetes, and obesity. Through murine, primate, porcine, and even aquatic models (e.g., zebrafish), several neurological, behavioral, cardiovascular, and oncological disorders are being understood while developing new therapeutic approaches. Nematodes and arthropods are some of the new alternatives for biomedical science; however, regardless of the species, many animal research studies show the vital role of animal models in advancing biomedical research.
Animal research is considered a key element in advance of biomedical science. Although its use is controversial and raises ethical challenges, the contribution of animal models in medicine is essential for understanding the physiopathology and novel treatment alternatives for several animal and human diseases. Current pandemics’ pathology, such as the 2019 Coronavirus disease, has been studied in primate, rodent, and porcine models to recognize infection routes and develop therapeutic protocols. Worldwide issues such as diabetes, obesity, neurological disorders, pain, rehabilitation medicine, and surgical techniques require studying the process in different animal species before testing them on humans. Due to their relevance, this article aims to discuss the importance of animal models in diverse lines of biomedical research by analyzing the contributions of the various species utilized in science over the past five years about key topics concerning human and animal health.
1. Introduction
The use of animals in scientific research is controversial [ 1 ]. However, the transformation of medicine from an art to a science can be mainly attributed to using a wide range of animal models [ 2 ], selected according to their functional and genetic characteristics for specific research lines [ 3 ]. Animal models contribute significantly to the advance of biomedical science through their meaningful contributions to our growing understanding of pathological and biological processes [ 4 ]. Moreover, they enable the development and testing of drugs, vaccines, and surgical techniques applicable to human and veterinary medicine [ 5 ].
The term “animal model” comes from the Latin animae (alma or spirit) and the word model, which means to imitate or be similar to [ 6 ]. Animal models are based on the principle of comparative medicine [ 7 ] as instruments that can replicate physiological and pathological processes [ 8 ]. The species is selected according to each project’s objective and hypothesis [ 3 ] but also considers biological, anatomical, functional, and genetic similarities to humans or other animals [ 6 ]. Today, most of the species utilized in biomedical research are rodents [ 9 ], as they are deemed ideal models for studying pathologies that affect human populations due to their physiological homology [ 10 ], which allows them to be employed to further our understanding of such processes as sepsis, obesity, cancer, organ transplants, and biological development, among many others [ 11 , 12 ].
The species used in experimentation are not limited to small mammals. Rhesus monkeys ( Macaca mulata ) are utilized to study high-priority diseases such as the pandemic caused by the severe, acute respiratory syndrome type 2 coronavirus (SARS-CoV-2) [ 13 ]. Domestic pigs ( Sus scrofa ) are crucial for organ transplant medicine and immune therapies [ 14 ]. New species, including some invertebrates such as fruit flies ( Drosophila melanogaster ), are used to study neurological disorders such as epilepsy [ 15 ], nematodes such as Caenorhabditis elegans to study obesity [ 16 ], and aquatic models, such as the zebrafish ( Danio rerio ), to treat metabolic disorders, including diabetes [ 17 ].
The broad range of species used in research has brought exponential advances in medicine, especially with the introduction of genetically modified (transgenic) animals [ 18 ] and the implementation of supporting technologies such as nanotechnology and artificial intelligence [ 19 ]. In light of this, this article aims to discuss the importance of animal models in diverse lines of biomedical research by analyzing the contributions of the various species utilized in science over the past five years concerning key topics of human and animal health.
2. Search Methodology
The literature search was performed in the Web of Science, Scopus, and PubMed. Keywords related to the use of animal models applied to current research priorities were searched to select the relevant articles, for example, “emerging infectious disease”, “diabetes and obesity”, “neurodegenerative diseases”, “pain therapies”, “surgical techniques”, “cancer models”, and “alternative animal models”. The search was limited to articles published in English in the last five years (2019–2023) and related to human and non-human medicine and therapeutics.
3. A Review of Animal Experimentation
Animal models are essential for several biomedical research fields such as cancer biology and therapeutics, neuroscience, pharmacology and toxicology, neurobiology of diseases, endocrinology, public health, palliative medicine, also, in studies in human and animal biology and for the discovery and testing of new drugs, vaccines, and other biologicals (e.g., antibodies, hormones) whose validation requires preclinical studies in animals [ 6 , 20 ]. Currently, these models address current research priorities, considered as those imposing major global threats to human and animal health. These include diseases that have afflicted humankind or increased exponentially in recent years such as SARS-CoV-2, different types of cancer and their therapy, cardiovascular diseases, metabolic and neurodegenerative disorders, and experimental refinement of surgical techniques to treat these issues [ 21 ]. The models may involve complete animals or only particular cells, tissues, organs, genes, or other agents that reproduce pathological processes ( Figure 1 ) [ 8 , 22 ]. Species include rats, mice, guinea pigs, dogs, rabbits, birds, ruminants (cows, sheep), horses, fish, frogs, monkeys, cats, reptiles, squid, crabs, bees, chimpanzees, hamsters, sea slugs, pigs, nematodes (roundworm), fruit flies, and protozoans, among others [ 7 ].

Classification of various animal models. The animals used in science can be divided into five broad types. ( a ) The main ones are models in which animals are induced to present a pathology similar to one that affects humans or other animals by administering drugs or other biologicals, inflicting injuries, or subjecting them to stress or other environmental conditions. In contrast, models based on spontaneous changes ( b ) include animals where the normal course of their life predisposes them to develop a specific disease. ( c ) Genetically-modified test subjects are animals with knockin or knockout genes or proteins. In contrast to using healthy animals ( e ), negative models ( d ) employ individuals that are not susceptible to certain diseases but serve to evaluate susceptibility to a specific pathology. TBI: traumatic brain injury.
The importance of animals in medical science is reflected, for example, in the percentage of Nobel Prizes studies in Physiology or Medicine using animal models (90%) [ 5 ]. From 1901 to 2020, two-thirds of those awards (186 of 222 projects [ 7 ]) employed animal models to understand pathogenic mechanisms, metabolic diseases, diagnostic and therapeutic procedures, develop vaccines, or test the efficacy of novel drugs [ 22 ]. At least 144 species used in those animal-based studies were mammals, and 42% were rodents [ 7 ]. Dogs were the first animal model used in metabolic research on gastric secretions [ 23 ] and for discovering insulin [ 24 ]. To date, rodents are the predominant species in research ( Table 1 ) [ 9 ]. However, non-mammal species are trending, and the number of animals depends on the country and its legal regulation regarding the use and reporting of animals in research. Moreover, in some countries, there is no official annual report on animal research (e.g., South America), and not every country counts the same animals (e.g., the United States does not consider rats, mice, fish, birds, amphibians, reptiles, and cephalopods, they are not covered by the Animal Welfare Act). Although it might differ, Table 1 and Table 2 show an overview of the use of animals according to species in some countries and a summary of the reported statistics worldwide.
Overview of the number of animals used in research, according to the species.
1 Excluding Northern Ireland; 2 Rats, mice, fish, birds, amphibians, reptiles, and cephalopods are not included.
Approximate of the number of animals used in research worldwide between 2019–2020.
Several Nobel Prizes have been awarded for animal research, and the increasing number of animal models in different countries demonstrates these studies’ importance for scientific advancement [ 7 ]. However, just as necessary, their use also entails ethical challenges that require surveillance through laws, norms, guides, and strict bioethical committees to monitor the use and care of laboratory animals based on the principles of the 3Rs [ 33 ]. In this regard, for 50 years, the National Center for the Replacement, Reduction, and Refinement of Animals in Research (NC3Rs) has promoted Russel and Burch’s initiative of the 3Rs to reduce, replace, and refine procedures to improve the conditions of animals used in experimental protocols [ 34 ].
These norms differ from one nation to the next. However, one guide recognized internationally is ARRIVE (Animal Research: Reporting of in vivo Experiments), developed in 2010 to improve the in vivo experiments description to increase the reproducibility of results, refine the stages of study design, and clearly report the methods so they can be repeated and tested [ 35 ]. A second guide is PREPARE (Planning Research and Experimental Procedures on Animals), which seeks to determine and guarantee quality control in animal studies [ 36 ]. Today, for any experimental protocol requiring animals, proposals such as the Animal Study Registry (ASR) help researchers thoroughly plan their study design, methods, and statistical analyses to ensure transparency and reproducibility in their results [ 37 ]. Additionally, it is essential to mention that Ethic Committees must approve current experimental protocols within each institute to promote an appropriate use and care for animals in research.
Animal models certainly provide valuable information on the nature of diseases [ 38 ]. However, it is important to remember that inter-species limitations exist in anatomy, metabolism, physiology, and genetics [ 39 ], so a single preclinical model cannot represent all aspects of pathogenesis due to differences in resistance or susceptibility [ 38 ]. Currently, many animals used in biomedical studies undergo some genetic modification, such as transgenesis or the utilization of knockout or knockin genes, to visualize specific changes that would take years to develop under normal conditions [ 40 ]. Therefore, the selection of the animals depends on the specific research field; through their use, researchers develop scientific knowledge focused on human and veterinary medicine.
4. Animal Models and Their Application in Distinct Fields of Current Biomedical Science
4.1. emerging infectious diseases.
The SARS-CoV-2 virus is the etiologic agent of the coronavirus 2019 disease (COVID-19) [ 41 ]. This disease has claimed the lives of over 6.3 million people worldwide since 2019 [ 42 , 43 ]. The lack of knowledge of this virus and its rapid propagation at the onset of the pandemic made it essential to determine its physiopathology and identify therapeutic agents and vaccines that could mitigate its threatening consequences. These fundamental issues were solved using in vivo assays that replicated the virus in animals to untangle its pathogenesis, the immune response, and the adverse effects that might result from the vaccines and therapies proposed before testing in humans and their release to the public [ 41 , 44 ].
The choice of an animal model that would allow researchers to observe the histopathological, radiological, or immune changes that the virus caused required that the test animals be susceptible to lung tissue damage and capable of developing an inflammatory process [ 45 ]. Potential species included nonhuman primates, ferrets, rats, mice, Syrian hamsters, lagomorphs, minks, cats, camelids, and even zebrafish [ 46 ].
The transgenic mice can express the human angiotensin-converting enzyme II (hACE2), a functional receptor for the SARS-CoV-2 virus that mimics clinical signs observed in humans [ 47 ]. Sun et al.’s [ 48 ] research with 4.5–30-week-old transgenic mice successfully replicated the virus after intranasal and intragastric inoculation. It led to the discovery of viral loads in the lung, trachea, brain, and feces. Those authors also detected an immune and inflammatory response due to the presence of interleukins (IL). Adult mice showed more lesions in the alveolar epithelial cells, focal pulmonary hemorrhage, and more significant apoptosis of macrophages. Those findings concurred with human reports showing that COVID-19 affected older adults more severely, with the over-65 population representing 80% of all hospitalizations and a 23-fold greater risk of mortality. Reports emphasized clinical signs, such as respiratory distress and cytokine release syndromes [ 49 ]. Studies with Syrian hamsters found that while the virus is lung-tropic and infects the respiratory tract by binding to the ACE2 cell surface in the alveoli, causing pneumonia in 67% of the animals, the gastrointestinal signs reported in humans are due to viral replication and dissemination in enterocytes [ 50 ].
One animal model that shares multiple similarities with humans for the physiopathology of the SARS-CoV-2 virus is based on Rhesus macaques, African green monkeys ( Chlorocebus aethiops ), and crab-eating macaques ( Cynomolgus macaques ) [ 51 ]. The latter has been utilized to replicate the infection conditions in young (males and females of 3–9 years) and old-aged animals (23–29 years-old females). After intranasal and intratracheal viral inoculations, researchers found that nasal swabs (peak viral load of 10 6 copies/µL) had higher viral loads than pharynx and rectal ones (a maximum of 10 4 copies/µL). Additionally, viruses from nasal and pharynx samples were detected for longer periods in elderly monkeys [ 52 ]. This relation between age and disease mortality was also reported in Rhesus monkeys. Comparative studies of three nonhuman primates (three 3–5 years and two 15 years old macaques) infected intratracheally revealed that the viral replication detected by nasopharyngeal and anal swabs was persistently detected from 3 days post-infection (dpi) to 11 dpi in elderly animals. In older macaques, 104–107.5 copies/mL were also detected (while young individuals had approximately 104 copies/mL), often accompanied by the development of diffuse severe interstitial pneumonia [ 53 ].
The reinfection processes prevalent in human populations were replicated in studies with C. aethiops . Infection in six animals caused signs such as fever (50%), hypercapnia (66%), 2–7-fold increases in C-reactive protein concentrations (100%), and coagulopathy (100%) were recorded. That research proved that anal, oral, and nasal swabs could detect viral loads up to 15 dpi [ 44 ]. These findings are similar to those from other works with M. mulata , where viral RNA was found in swabs from the nose, pharynx, and anus, with amounts increasing up to 3 dpi (in an approximate range of 4–7 copies/mL) [ 53 ]. These nonhuman primate models undoubtedly contributed significantly to our understanding of the pathogenicity of COVID-19 and the physiological bases for implementing preventive and diagnostic measures and treatment.
Another important aspect of using animals is that they helped understand the transmission of the virus to other domestic species and showed that pets could acquire the SARS-CoV-2 virus through contact with an infected human. However, there is no evidence of active pet-to-human transmission [ 54 ]. Studies with dogs, pigs, chickens, and ducks showed they were not susceptible to COVID-19 infection due to low viral replication [ 55 ]. Identifying susceptible species made it possible to choose appropriate models for developing and testing vaccines [ 55 ]. Ferrets, Syrian hamsters, rabbits, transgenic mice [ 47 ], and cats were all found to be susceptible, the latter even vulnerable to airborne transmission with the development of clinical signs such as hair loss and pulmonary alterations similar to those seen in humans [ 56 , 57 ]. Apart from domestic cats, wild felines (tigers, lions, pumas, snow leopards) [ 58 ] have been reported to show infections by this virus. Kang et al. [ 59 ], who reported the first Delta variant (SARS-CoV-2 Delta) case in three domestic cats with COVID-19-positive owners in China, insist that transmission to pets is a topic of concern due to their possible role as silent intermediate hosts.
4.2. Endocrinology and Metabolic Pathologies
Obesity is a public health problem affecting over 600 million people worldwide [ 60 ]. Obesity and its associated metabolic syndromes have consequences such as knee osteoarthritis, a disease prevalent in approximately 60% of the overweight population [ 61 ], but this is also associated with cancer, cardiovascular disease, hypertension, coronary artery disease, stroke, sleep apnea, asthma, gallstones, steatohepatitis, and dyslipidemia. Over one-third of the world’s overweight or obese population is at risk of developing type 2 diabetes mellitus [ 23 ]. Using rodent models, researchers have determined that one element that promotes the development of type 2 diabetes mellitus is adipose tissue inflammation due to insulin resistance and excess fat mass [ 62 ]. The increase in the presentation of these comorbidities has led to the use of animal models to test new, improved strategies for reducing the incidence of this disease.
The role of the different types of adipose tissue in humans and animals is a crucial line of research that has developed with the use of rodents. For example, adipogenesis suppression and the browning of white adipose tissue (WAT) [ 63 ] have been suggested as strategies for preventing obesity [ 60 ]. The browning process creates a brown adipose-like tissue (BAT) that can participate in thermogenesis by transforming caloric intake into heat [ 64 ]. Since this is part of a central nervous system response to cold, certain medications and exercise can trigger browning as has been observed in obese and lean rats subjected to high-intensity training. In C57BL/6J mice, the transformation of beige adipocytes into WAT can be promoted with diets complemented with resveratrol for 16 weeks, as this induces a change in the intestinal microbiota in treated animals ( p < 0.01) (increasing microorganisms of the genera Bacteroides , Lachnospiraceae , Blautia , Lachnoclostridium , and Parabacteroides , among others) that modulates lipid metabolism and has anti-inflammatory properties and anti-obesity effects [ 65 ].
The importance of physical activity in treating these conditions has been demonstrated in experiments with 48 Sprague-Dawley male rats, where aerobic exercise for 12 weeks combined with prebiotic fiber supplementation prevented knee joint damage, dyslipidemia, endotoxemia and normalized the effects of insulin resistance ( p < 0.001) [ 61 ]. Studies with these supplements as part of a therapeutic protocol in Wistar rats, administered in presentations such as yogurt, have shown that supplementation with 5% of yogurt reduces levels of oxidative stress (significant decreases in NO levels, p < 0.05), and had fewer amounts of inflammatory cell infiltration and collagen deposits in the liver ( p < 0.05) when compared to animals fed high-fat diets. According to these studies, this supplement could be a potential human therapeutic option [ 66 ].
Studies of the human genome have identified hundreds of genetic variants associated with obesity and opened the way to examining these genes in species such as C. elegans , a nematode capable of storing fat in the form of lipid droplets inside hypodermal and intestinal cells. C. elegans has 14 genes that promote diet-induced obesity and three that prevent it [ 67 ]. Those genes are now recognized as potential targets for anti-obesity treatment. Ke et al. [ 68 ] found that the knockdown of 23 fat-storing not only reduced excessive fat accumulation but also improved the health and lifespan of this species ( p < 0.05). The inhibitory effect of flavonoids such as butein on lipogenesis in C. elegans succeeded in reducing triglyceride levels by up to 27% without altering food intake or energy expenditure, an effect due to the downregulation of proteins involved in lipid metabolism [ 69 ]. Likewise, the appetite suppressant effect of administering vegetable extracts from the Lentinus strigosus mushroom (300 and 1000 µg/mL) to C. elegans functioned as a natural means of preventing obesity [ 70 ]. Studies of this kind allow researchers to address obesity as a complex pathology affected by diverse factors: diet, physical activity, developmental stage, age, genes, and environmental interaction [ 67 ].
Another animal species considered a promising model for studying metabolic syndromes is the zebrafish ( D. rerio ). This species has genetic homology with humans, so through genetic mutation, chemical induction, and changes in diet, they can be used to study hyperglycemia, obesity, diabetes, and hypertriglyceridemia [ 71 ]. Pigs, meanwhile, share similarities with humans in terms of organ size, lifespan, anatomy, physiology, and metabolic profile [ 40 ]. A study of obesity in Iberian pigs showed the pathogenesis of chronic kidney disease caused by overweight and obesity. Although the administration of high-fat diets did not generate diabetes in those pigs by day 100, analyses revealed hypercholesterolemia (142 ± 27 mg/dl), hypertriglyceridemia (75 ± 43), insulin resistance, and glomerular hyperfiltration [ 72 ]. These effects also occur in humans [ 73 ] and have been studied in obese male mice and ovariectomized females [ 74 ].
The domestic dog has been postulated as a valuable model for studying chronic morbidities brought on by environmental conditions since they share morbidity and mortality factors with humans. In this field, Hoffman et al. [ 75 ] reported that comorbidities behind chronic conditions such as obesity, arthritis, hypothyroidism, and diabetes reported in humans were also present in 73,835 canines and that those dogs showed a positive association between age and the number of morbidities ( p < 0.001). Other studies have revealed that obesity in dogs (137/198) is closely linked to the alimentary habits of their owners, finding that the 79.8% of dogs from overweight owners (114 persons) were obese ( p < 0.001) [ 76 ]. Therefore, studies of these animals could provide information on disease interaction.
4.3. Cancer in Biomedicine
According to the World Health Organization [ 77 ] and the National Cancer Institute [ 77 , 78 ], the most common types of cancer in humans in 2020 were breast (2.26 million cases), lung (2.21 million), colorectal (1.93 million), prostate (1.41 million), skin (1.20 million), and stomach (1.09 million). These cancers cause 10 million deaths per year. Projections for 2022 estimate that around 1,918,030 new cancer cases will be diagnosed in the United States, with 350 cancer-induced deaths per day, making this disease a primary cause of mortality [ 79 ]. The pathogeny of these cancers and testing new treatment options is another field that extensively uses animal models. Over 95% of studies use rats and mice to inject cancer cell lines subcutaneously, study the primary cancer lesion, and follow its growth before excising tumors [ 80 , 81 ]. However, one disadvantage of this subcutaneous tumor model, is that injections in athymic nude mice may not accurately represent the interaction among tumor cells, local stroma, and the tumor’s microenvironment, depending on its precise location [ 82 ]. Contrarily, orthotopic murine models have been shown to replicate the tumor microenvironment –including metastasis– when inoculated in the original anatomical site of the tumor. In female BALB7c mice, inoculation of mammary cancer cell line 4T1 as a fat pad tumor model showed that 50% of the animals had metastasis to the ovaries, spleen, liver, and sternum. However, when compared to a heterotopic model, orthotopic tumors were smaller (1993.7 ± 197.15 mm 3 vs. 1078.4 ± 300.26 mm 3 , p < 0.05) and had a significantly lower percentage of infiltrating cells ( p < 0.05) [ 83 ]. Moreover, these orthotropic models, together with in vivo optical metabolic imaging, are proposed as an approach to studying how, for example, the fatty acid uptake by breast cancer cells increases accordingly to tumor aggressiveness and metastatic process ( p < 0.05) [ 84 ] Attacking this complication in tumor development is the principal objective of anticancer therapies, since most deaths from prostate cancer, for example, are due to metastasis into bone structures [ 80 ].
Koosha et al. [ 85 ] used diosmetin, an anti-tumorigenic, in colon cancer xenografts in 24 male nude mice. Results showed that tumor volume in the group treated with 100 mg/kg of diosmetin was significantly smaller than in the untreated group (264 ± 238.3 vs. 1428 ± 459.6 mm 3 , p < 0.01). Promisingly, the drug did not produce toxicity even when administered at high doses. Studies of this kind show that laboratory animals allow researchers to test new drugs and better understand disease development but also aid in determining non-toxic doses that can be applied to humans or animals. Using these models as translational media for studying cancer has also revealed the importance of identifying the pain that animals may experience. Pain assessment is important in in human medicine and laboratory animal welfare. In this regard, recognizing degrees of cancer-induced bone pain has been studied by observing behavioral changes in rats and mice, where innate behaviors, such as burrowing, are reduced 9 days after inoculation when compared to control groups ( p < 0.05) as a result of the nociception associated with the degree of severity of cancer due to reduced bone density [ 86 ].
The fact that the canine and human genomes share a high degree of similarity (75%) and that the risks of death due to neoplastic, congenital, and metabolic diseases are comparable means that the dog is an ideal translational model for studying human morbidity and mortality [ 75 , 87 ]. For example, the percentage of neoplasia is similar between dogs and humans (27.4 vs. 25.3%). However, because the types of cancer that affect each species correlate only marginally (Spearman rank p = 0.661) [ 75 ], dogs have been replaced in many preclinical studies by genetically-modified pigs [ 87 ].
Another novel anticancer strategy involves managing nerve-tumor interaction [ 88 ] since tumor-specific denervation can suppress neoplasia growth [ 89 ]. A study by Kamiya et al. [ 90 ] with female Balb/c-nu mice and the use of xenografts in Hras128 rats in a model of chemically-induced breast cancer showed that sympathetic stimulation of the nerves in tumors accelerated cancer growth but that parasympathetic stimulation reduced growth and downregulated the expression of programmed death. In contrast, in the case of late-stage colorectal cancer, parasympathetic denervation via vagotomy and atropine administration in 150 male Wistar rats reduced the incidence of tumors and their weight and volume after eight weeks, as well as cell proliferation, angiogenesis, and regulated expression of the nerve growth factor [ 89 ].
These neural anticancer therapies in humans and animals indicate that while sympathetic nerves show cancer-promoting effects in prostate and breast cancer, and melanoma cases, the parasympathetic/vagal nerves are believed to trigger both reactions. For example, vagal nerves can promote prostate, gastric, and colorectal cancers, but suppress breast and pancreatic cancers, due to β-adrenergic and muscarinic effects that modify the behavior of cancer cells, angiogenesis, tumor-associated macrophages, and antitumor immunity [ 88 ]. The axonogenesis process in species such as mice, linked to the development of metastasis in breast cancer, showed through immunofluorescence that nerve twigs tend to be sympathetic-like, with no expression of parasympathetic fibers [ 91 ].
In addition to the support of laboratory techniques such as immunofluorescence, non-invasive diagnostic methods are a priority in oncology. In immunocompetent genetically-engineered mouse models, Kirkpatrick et al. [ 92 ] utilized nanosensors with urine tests to detect protease activity in diverse types of cancer, including lung cancer, achieving 100% specificity and 81% sensitivity. In this way, monitoring with nanosensors and clinical assays in animals has demonstrated that this technique can be an option for conducting accurate, radiation-free diagnostic tests.
Nanoparticles and their application, together with in vivo imaging, can help to test novel luminescent particles and assess their tissue penetration to improve cancer therapy [ 93 ]. In vivo imaging enables us to understand tumor growth-related processes such as oxidative mitochondrial metabolism in mouse models with cell lung cancer [ 94 ]. Likewise, in a mouse model of brain tumor –glioblastoma– under general anesthesia, modified in vivo optical imaging (Surface enhanced spatially offset Raman scattering) covers the inability of conventional techniques that rely on subcutaneous inoculation of cancerous cells because they cannot read deep tissues [ 95 ]. These techniques are the basis for imaging-guided phototherapies that are a current research field to find agents capable of inducing tumor cell apoptosis, such as photodynamic y and photothermal therapy [ 96 ].
4.4. Pharmacology and Therapeutics
Parallel to the advances in our knowledge of the physiopathology of diverse conditions, developing and testing new therapeutic options is another field destined for animal models. Algology is a science in constant actualization to provide new and efficient drugs to prevent the consequences of pain by reducing the number and severity of secondary effects in both human and veterinary patients [ 97 , 98 ]. Adequate models are needed to evaluate analgesic efficacy accurately. In the case of treatments for open wounds, Parra et al. [ 99 ] applied carprofen (5 mg/kg) and buprenorphine (0.1 mg/kg) to the left hind paw of Sprague Dawley rats of both sexes using a punch biopsy to assess analgesia in an open wound model. Using four behavioral tests associated with aspects of nociception, mechanical and thermal stimulation, guarding behavior, and the weight-bearing test, they found that carprofen promoted recovery of the thermal response to basal levels after just 2 h. The same rat species were utilized to test the renal and gastrointestinal safety of non-steroidal anti-inflammatory drugs (NSAIDs) such as ibuprofen by administering single and multiple oral doses to pediatric patients. Furthermore, the necropsies performed on pigs of different ages (8-week-old and 6-to-7-months-old) in the study by Millecam et al. [ 100 ] revealed no severe lesions in the stomach after multiple doses of ibuprofen at 5 mg/kg. However, significant histological score differences ( p < 0.025) were observed in the duodenum (1.38 vs. 4) and jejunum (3.63 vs. 1.25) between the experimental and control group. Additionally, an increased clearance time for the drug after multiple doses was found, an effect similar to reports in human pediatric patients.
Due to the adverse effects that NSAIDs can generate, especially for treating chronic afflictions such as arthritis and cancer, opioids are another therapeutic option [ 101 ]. However, since the long-term use of these drugs is also associated with complications, research has begun to new concepts and explore directions. The opioid-free anesthesia technique was introduced to prevent tolerance and hyperalgesia and reduce the use of these drugs in the postoperative period. This method uses agents such as alpha-2-agonists, ketamine, and local analgesics with distinct action mechanisms in multimodal analgesia [ 102 , 103 , 104 ]. Other new opioid-based pharmacological options are transdermal patches impregnated with morphine-like compounds. In 6–12-week-old C57BL/6JJmsSlc mice, patches synthesized with two new opioids (new-opioids 1 and 2, N1 at 3 mg/kg; N2 at 10 mg/kg) showed the same analgesic efficacy as morphine at 3 mg/kg. The effect remained constant, even under repeated administration (in contrast to fentanyl), and the cutaneous trans-permeability rate was greater, at 1.71 ± 0.35 and 3.94 ± 1.36 µg/cm/h [ 105 ]. The administration of opioid nanoparticles has also been suggested to prevent opioid tolerance and reduce the severity of adverse effects. Leucine-enkephalin hydrochloride-based nanoparticles with a size of 100–200 nm have been tested in male Sprague Dawley rats by applying them intranasally, reaching the brain directly. After dosing, high concentrations were found in the olfactory bulb and cerebrum between the first 60 min (approximately 80 ng/g and 160 ng/g, respectively), while plasma concentrations were not detected at any evaluation time ( p < 0.0001). This prevents the side effects of drug transit through peripheral pathways [ 106 ].
Techniques based on local anesthesia temporarily relieve pain by inhibiting nerve impulse transmission. However, when used to complement multimodal analgesia protocols, they can be associated with neurotoxicity in both human and veterinary patients [ 107 ]. Administration via polymer-based encapsulation is a new strategy designed to prevent toxicity and permit the prolonged release of the active ingredient to give a long-term analgesic effect for up to seven days [ 107 ]. A ketamine-polymer-based drug was applied transdermally to Wistar rats to determine its analgesic effects [ 98 ]. Results of the tail-flick test and readings from an analgesiometer led them to determine a significant analgesic effect ( p < 0.01) maintained for 24 h with a peak effect at 8 h and a response time on the test 5.72 s vs. a basal time of 2.44 s. The compound did not produce irritation when tested on rabbit skin. It prevented the secondary effects of intravenous, nasal, or oral administration, so it is a potential option for treating neuropathic pain [ 108 ].
4.5. Experimental Surgical Tecniques
In addition to developing novel drugs, advances in surgical technology and techniques have opened fields in microsurgery in human and animal medicine since the 1900s when Carrel and Guthrie performed the first transplants in dogs [ 109 ]. Later, in 1950–1960, Buncke and Schultz tested the first microsurgery techniques using models of digital amputations and reimplantation in Rhesus monkeys, performing vascular microsurgery to restore circulatory connections successfully [ 110 ]. Anastomosis of 1-mm blood vessels in the ears of adult rabbits by reimplantation was the first demonstration of microsurgery in reconstructive medicine [ 111 ].
Today, rodents are considered models for reimplanting extremities and restoring blood vessels because their vascularization is homologous to the human finger [ 112 ]. For example, developing heterotrophic osteomyocutaneus flap transplant protocols in Lewis rats furthered our understanding of the mechanisms and pathways involved in the immune response underlying tissue transplant rejection [ 113 ]. Likewise, in an experiment with five syngeneic mice and allografts—using a donor-supplied aorta and inferior vena cava—end-to-end anastomosis of those structures showed a 74% success rate as a technique for hind limb transplants [ 114 ]. In another study, Tee et al. [ 115 ] performed grafts of engineered cardiac muscle flaps in the epicardium of 8 rats. The flaps were transplanted by microsurgery to resolve one of the first limitations: failed vascular anastomosis. Those researchers performed successful end-to-end anastomosis of the carotid artery and jugular vein by placing the flap on the epicardium, achieving a survival rate of 75% during 4 weeks post-surgery, with viable cardiomyocytes and vascular connections between the flap and the epicardium by week 10 [ 115 ]. These techniques, tested first in animals, were later used with human patients with coronary artery disease caused by diseases such as squamous cell carcinoma, with a 96% survival rate of the flap in individuals subjected to neck and head surgery [ 116 ].
Another advance in biomedicine achieved thanks to experimental work with animal species such as pigs are based on animal-to-human organ transplants. On 7 January 2022, Bartley Griffith’s team performed the first heart transplant from a genetically-modified pig to a 57-year-old human patient with terminal heart disease [ 117 ]. Although the patient’s condition who received that xenotransplant deteriorated two months after surgery, and he died, the procedure set an important precedent. It showed the need to continue research on genetically-engineered animal organs and immunosuppressor drugs since the immune response and organ rejection are still the leading causes of transplant failure, especially when the organs come from other animal species [ 118 ].
Due to the physiological similarity between nonhuman primates and humans, procedures for organ transplants are often tested in those species. Over seven years, Lee et al. [ 119 ] performed 22 xenotransplants using hearts from transgenic pigs eliminating alpha-galactosidase transferase knockout or expression of the regulatory proteins CD46, CD39, or CD73 in Cynomolgus monkeys ( Macaca fascicularis ). Results showed that survival of the grafts was significantly higher in hearts with double or triple genetic manipulation (11.63 ± 11.29 days vs. 30.83 ± 20.34 days, p = 0.03). This is similar to the report by Cui et al. [ 120 ] on triple knockout cells from pigs (that do not express any of the three carbohydrate xenoantigens). The complement-dependent cytotoxicity response and the amount of anti-pig IgG/IgM immunoglobulins (Ig) were evaluated in serum from 72 specific pathogen-free (SPF) baboons and in human serum. Results for humans and old-world monkeys showed similar antibody binding, but the cytotoxicity measured in IgM and IgG was lower in the humans ( p < 0.05 vs. p < 0.01).
Observations on the immunosuppressor response to compounds such as anti-thymocyte globulin (20 mg/kg) and rituximab (20 mg/kg) demonstrate that, in addition to the use of transgenic animals, a strict immunosuppressor regimen is a critical element in allotransplants [ 119 ]. In this regard, drugs injected in nanoparticles such as mycophenolate mofetil allow low-water soluble compounds to be combined with other compounds and administered as solid lipid nanoparticles to improve their absorption and release by as much as 68% in acid media [ 121 ].
In this field, sustained release options such as nanoparticle-anchoring hydrogel scaffolds of the immunosuppressant tacrolimus allowed the localized release of the drug with tissue regeneration in nude female mice or those of the BALB/c line that were given the drug in the hind limb. Those combinations allowed the sustained release of 77% of the drug, without toxicity, within 28 days at <100 ng/mL [ 122 ]. Thus, refining these drugs in the future will make it possible to reduce the cases of organ rejection due to the immune response. This finding is significant because their benefits are not accompanied by systemic toxicity, complications, or dose reduction without pharmacological efficacy [ 123 ].
4.6. Neurosciences
The field of neuroscience includes surgical and therapeutic procedures involving the central nervous system and conducts studies focused on specific diseases or pathologies of that system. With the discovery of neurological sequelae in COVID-19-infected patients, animal models have allowed researchers to observe the effects that the SARS-CoV-2 virus generates in sporadic cases, including epileptic seizures and encephalitis with a mortality rate of approximately 5.3% [ 124 ].
Estimates suggest that approximately 42 million people worldwide suffer brain injuries annually and that 80% of cases are classified as traumatic brain injury (TBI). Animal models based on rodent species are being used to improve our understanding of the physiopathology of TBI [ 125 ], though authors such as Vink [ 126 ] caution that neuroanatomical differences in the mouse’s lissencephalic brain can generate biomechanical responses distinct from those in humans. Moreover, the replication of trauma may be greater in rodents since traumatisms in these animals tend to generate focal instead of diffuse lesions [ 127 ]. Grovola et al. [ 128 ] used male Yucatán miniature pigs to analyze neurological dysfunction in animals with mild traumatism 1-year postevent. They found a persistent neuroimmune response in animals with morphological changes to the microglia, with increased branches and junctions per cell ( p = 0.026 and p = 0.045, respectively). In other research, models of medullar lesions are widely utilized with species such as rats, which are particularly important because between 236 and 1009 per million humans annually suffer a spinal cord injury [ 129 ]. Although this species is the one most often employed to replicate medullar damage, Filipp et al. [ 129 ] affirm that between-species differences (quadrupeds, bipeds) must be considered when evaluating the neuroplasticity of the spinal neurons.
Epilepsy is one of the most common neurological conditions, affecting over 50 million people worldwide [ 130 ] and 0.6–0.75% of the domestic canine population [ 131 ]. Recent studies of the physiopathology of this disorder and the testing of anti-seizure drugs have used fruit flies ( D. melanogaster ) because they manifest seizure-like behavior and share 70% of their genes with humans [ 15 ]. The use of the endocannabinoid anandamide (at 2, 20, and 200 µg/mL) in Drosophilas prevented induced seizures ( p < 0.0001). This led to the discovery that the action mechanism of their metabolites is not linked to the cannabinoid receptors but, instead, to transient potential receptors (TRP). This makes the fruit fly a suitable medium for studying this type of drug [ 132 ].
Despite its nature and supposed organic simplicity, Drosophila has been used to understand the neurobiological bases of processes still considered mysteries by biology, such as sleep, plasticity, and memory [ 133 ]. After studying 12,000 exemplars of D. melanogaster , Toda et al. [ 134 ] reported the existence of the “nemuri” gene, a peptide with antimicrobial properties that favors sleep and helps these flies survive the infection. This suggests that its function could be linked to the immune competence of the sleep process in animals and humans. The association of sleep with long-term memory, known as post-learning sleep, was studied by Lei et al. [ 135 ], who found a neural circuit that excites the mushroom body neurons and a connection to the fan-shaped ventral neurons that promotes post-learning sleep during courtship. This finding underlined the association between the longer learning experience and the reinforcement of long-term memory, mechanisms sometimes found in mammals.
Neuroscience techniques applied to species such as nonhuman primates and transgenic models of those species have recently been proposed as useful for studying human evolution and the cerebral functioning of people with autism disorders and neurodegenerative diseases such as Alzheimer’s [ 136 ]. In humans, Alzheimer’s disease is considered the most common neurodegenerative disease accounting for around 80% of cases of dementia worldwide [ 137 ]. It is widely recognized that mitochondrial dysfunction is an event that precedes the onset of Alzheimer’s, and this has been studied in two lines of mice (APPswe/PSEN1 ∆E9 and C57BL/6J). There, the alteration of mitochondrial homeostasis and increased mitochondrial calcium levels caused damage and neuronal death ( p < 0.0001) due to deposits of amyloid plaques. Recognition of this physiopathology helped scientists establish the goal of preventing this process as a novel therapeutic approach [ 138 ].
Another neurogenerative disease, Parkinson’s, has been studied primarily with murine models [ 139 ]. Recently, however, researchers recognized that the zebrafish shares more neuroanatomical traits with humans and that mutations of the PARK7 gene in adult fish were associated with the development of Parkinson’s in humans [ 140 , 141 ]. Exposure of zebrafish larvae to neurotoxins that act directly on the dopaminergic neurons constitutes a method to mimic the phenotype of Parkinson’s disease. Specifically, the MPP+ neurotoxin affected the locomotor function (total distance and velocity) of fish, reducing its performance by 80% and 85%, respectively ( p < 0.001). Furthermore, no systemic effects were observed, presenting a condition similar to Parkinson’s [ 142 ].
Palliative treatments to control movement disorders such as dystonia, Huntington’s, and Parkinson’s disease have also been tested in zebrafish [ 143 ]. Treatment of Parkinsonian embryos with substances such as rosmarinic acid (RA) prevents the loss of dopaminergic neurons due to neurotoxicity. This acid has been proposed as a neuroprotector and antioxidant that reduces locomotor deficits measured, for example, by increasing the swimming distance in zebrafish treated with RA at concentrations of 10 or 100 µm (approximately 130 to 150 cm, p < 0.01) [ 144 ]. Similarly, it has been suggested that herbal medicines based on Tongtian oral liquid have neuroprotective and antioxidant properties. The administration of Tongtian to zebrafish prevented neurotoxicity and the degeneration of dopaminergic neurons ( p < 0.01 when compared to non-treated fish) while reducing larval behavioral impairment measured as improvements in the total distance (peak distance around 180 cm) and velocity (peak values around 3.5 cm/s) ( p < 0.001) [ 145 ].
Aquatic models are also utilized to study other neurodevelopmental problems, such as autism spectrum disorder in zebrafish and Medaka fish ( Oryzas celebensis ) [ 146 ]. Chen et al. [ 147 ] found that prenatal exposure to valproic acid (at 5 and 50 µM) in AB lines of zebrafish produced embryos and larvae with signs similar to those seen in autistic humans, including hyperactivity, manifested in a greater frequency of tail-bending, greater distances traveled after touching of the dorsal tail ( p < 0.001, p < 0.05), increased swimming speed under both light and dark conditions, and deficient social interaction, anxiety, and macrocephaly, all as consequences of neuronal cerebral cell proliferation. In a separate study, when applied to 28 neonate rat pups, this acid generated oxidative stress in the cerebellar hemispheres and reduced the count and nuclear size of the Purkinje cells [ 148 ]. These findings appeared, as well, in the brains of children with this condition. In the case of rats, administering grape seed extract served as a neuroprotector thanks to its antioxidant effect.
Referring to neurodegenerative disorders, a key strategy is to improve symptomatology through physiotherapy and rehabilitation protocols, another line of research that has increased in importance due to the prevalence of neurological conditions that can affect the quality of life of both humans and animals.
4.7. Physiotherapy and Rehabilitation
Because the number of neurodegenerative and traumatic diseases in humans and animals has been increasing in recent years, one of the main options for these cases is developing and implementing physiotherapy techniques. For example, stimulation of the lateral cerebellar nucleus with low-intensity ultrasound is a non-invasive therapy for reducing the consequences of cerebrovascular accidents in mice after induced ischemic stroke. In those test animals, functional asymmetry of the brain was restored, and pathological electrical cerebral delta activity was reduced, leading to improved performance on the beam-walking test [ 149 ].
In cases of osteoarthritis, for example, transcutaneous electrical nerve stimulation techniques (TENS) in physiotherapy protocols utilized in male Sprague Dawley rats with induced pain showed that when applied to the knee joint for 20 min a day for two weeks, TENS reduced the expression of c-fos ( p < 0.05) (a biomarker of pain) on the day following the intervention (7302.80 ± 152.40% vs. 5074.50 ± 199.50%) in all the test animals that, in addition to TENS, did exercise on a treadmill (7333.40 ± 156.70% vs. 2790.00 ± 111.88%) [ 150 ]. In canine patients, functional neurorehabilitation after Hansen type I intervertebral disc surgery has been tested using a technique with bases similar to TENS called transcutaneous electrical spinal cord stimulation (TESCS). Combined with pharmacological treatment (4-AP) for 90 days, this approach restored ambulation in 88% of 16 animals thanks to the so-called multimodal neurorehabilitation protocol in a study by Martins et al. [ 151 ].
In human medicine, TENS has been used with patients with knee osteoarthritis. It improved performance on the stair-climbing test by 0.41 s [ 152 ] and reduced pain in individuals with head and neck cancer who had received radiation and developed oral mucositis with the pain. In those patients, 30 min of high-frequency TENS functioned as a non-pharmacological intervention that reduced pain levels at rest by approximately 3.0 from visit #1 to visit #3, as measured by the McGill Pain Questionnaire. However, this approach did not show results for controlling functional pain [ 153 ]. Pain reduction allowed the patients to exercise the limb and prevent the loss of mass, muscular strength, and joint instability with some cartilage recovery.
Electroacupuncture is a similar technique used to control chronic inflammatory pain. The action mechanisms of this technique have been studied in murine models after administering the complete Freund’s adjuvant to the hind paw. In those animals, electroacupuncture produced analgesia by attenuating neuronal signaling in the dorsal ganglia of the spinal cord, the anterior cingulate cortex, and neurons of the somatosensorial cortex. This suggests that the analgesia generated affects cortical pain pathways and means that the somatosensorial and anterior cingulate cortices may be potential therapeutic targets for developing new options for pain management [ 154 ], one of the principal objectives of rehabilitative medicine in humans and animals.
5. New Models and Strategies Applied in Animal Research
The use of poorly developed or unconventional species is expanding to other areas of biomedicine. For example, the zebrafish is used to study anomalies in limbs and craniofacial regions [ 155 ]. In those fish, Bergen et al. [ 156 ] found 604 genes associated with processes of the formation, mineralization, and regeneration of scales, which demonstrated that those structures are reminiscent of bone. Mutations of these genes in humans generate bone mineralization disease. This suggests that scales could be a model for studying the pathogenesis of skeletal diseases, calcification, and matrix formation [ 156 ]. In another fish species –Medaka, the Japanese rice fish ( Oryzias latipes )– researchers found that the electrocardiogram pattern was more similar to that of humans than those of rats and mice. This led authors such as Yonekura et al. [ 157 ] to use it as a model for testing cardiovascular therapies and the response of action potentials to verapamil, which causes bradycardia, an effect also seen in humans [ 158 ].
In addition to the use of mammals such as domesticated dogs as models for research on urinary pathologies due to their anatomical and physiological similarity to humans [ 159 ], the diverse species that have been incorporated into biomedical science include protozoans, platyhelminths, planarians, cnidarians, bivalve mollusks, gastropods, cephalopods, annelids such as the tardigrades, and arthropods such as hexapods, crustaceans, arachnids, and various insects in studies in broad fields of investigation [ 160 ]. In dermo-cosmetology, extraction of hyaluronic acid from mollusks such as Mytilus galloprovincialis and Crassostrea gigas to treat wounds in Wistar rats accelerated the processes of wound repair and re-epithelization, allowing lesions to heal completely within 15 days of treatment, in contrast to the results attained with commercial healing creams [ 161 ]. Another application of a cephalopod ( Octopus vulguris ) is in reconstructive medicine due to its capacity to regenerate nerves and adjacent tissues such as muscle and blood vessels. Despite these technical advances s in medical research, additional studies are required to determine markers, antibodies, and imaging techniques designed to take advantage of those species [ 162 ].
Non-animal alternatives such as cell cultures, 3D tissue cultures or organs-on-chips, mathematical models, stem cells, bioprinting, in silico tests, and advanced computer simulations have been increasing in recent years [ 163 ]. In leading research countries and regions such as the United States, United Kingdom, China, Germany, Japan, Canada, and Australia, among others [ 164 ], there has been a particular interest in replacing animal models with another methods. This is promoted by ethical pressures, the 3Rs initiative, and official instances such as the National Institute of Health [ 165 ]. An example of this is the new US law sponsored by the Food and Drug Administration (FDA), which states that drugs no longer require animal testing before human clinical trials [ 166 ]. Another example could be Canada and the statistics regarding the number of rats and fish used as animal models from 2019 to 2020. In 2019, rats and fish went from 3.9% and 19.9% to 2.6% and 11.7%, respectively [ 26 , 167 ].
When mentioning tissue engineering, the so-called “organoids”—transplantable tissues created by engineering—have raised expectations for replacing animals, resolving specific bioethical issues by making the study of pathologies and drug testing more specialized [ 168 ]. Protocols for head and neck squamous carcinoma have been published, using patient-derived organoids to study therapeutic agents and their drug sensitivity [ 169 ]. However, as materials that depend on in vitro handling and do not come from organisms that provide blood flow or the biochemical conditions of a live individual, their development and clinical application require further advances, not only in medicine but also in applicable biotechnologies [ 168 ]. Current trials aim to establish the vascularization of organoids, such as in human brains [ 170 ] or kidney organoids., In vitro culturing under millifluidic chips and endothelial cells is an alternative to creating vascular networks that need future studies but can be an option to research nephropathies [ 171 ]. Complex vascular networks made with mesodermal progenitor cells by Wörsdörfer et al. [ 172 ] replicated the ultrastructure of a blood vessel in tumor organoids with endothelial cell junctions, luminal caveolae, microvesicles, and antiangiogenic responsiveness to stimuli. Moreover, 3D bioprinting of organoids derived from stem cells (e.g., ectoderm, mesoderm, and endoderm) is another alternative to replicate developmental diseases in the brain, skin, kidney, heart, intestine, lung, and liver [ 173 ]. Those biotechnological advances include approaches in which animal models are accompanied by artificial intelligence [ 174 ].
The support that robotics and artificial intelligence provide to the advance of science has improved the technologies involved in techniques of robot-assisted, minimally-invasive surgery [ 175 ]. Recently, machine learning techniques have been used with animal models to help diagnose or identify specific behavioral or physiological changes in species. In this regard, models of Parkinson’s disease in zebrafish have used video recording to teach the machine to differentiate between a movement disorder and a parkinsonian fish, a technique that may apply to cases of motor diseases in humans [ 140 ]. Deep learning algorithms, a type of machine learning, are another approach to the future of biomedical science, particularly in diagnosing a wide range of diseases. Based on CT images, it has been tested in hepatocellular carcinomas [ 176 ] and COVID-19 diagnosis, showing 85.2% accuracy a specificity and sensitivity of 88 and 87%, respectively [ 177 ]. A similar accuracy percentage (91%) was also obtained when testing deep learning to identify genetic syndromes according to facial features [ 178 ]. In veterinary medicine, automatizing facial recognition to assess pain is a current approach applied in cats, with an accuracy above 72% [ 179 ]. These applications suggest that new diagnostic tools might not require animal models. Nonetheless, implementing these technologies depends on their ability to simulate the physiology of a live organism, especially humans, to improve the replicability of results [ 180 ].
The replicability of animal models in preclinical protocols depends on their internal and external validity for transposing results to humans. However, the complexity of some human conditions and the physiological differences among species have led authors such as Pound and Ritskes-Hoitinga [ 181 ] to recommend focusing on techniques and technologies prioritizing human research. However, it is important to remember that experimentation with human subjects involves many serious ethical and legal controversies such as those surrounding experimentation with nonhuman primates [ 182 ]. One ethical way to deal with this topic consists in establishing and following norms and guidelines such as the 3R principles that promote the rational and humane use of laboratory species [ 33 ].
In summary, important advances in human and veterinary medicine have been mainly achieved thanks to animal species that allow us to improve our understanding of the etiology, pathology, physiology, and toxicology of diverse conditions that affect both humans and nonhuman animals [ 5 ]. However, using these species requires evaluating ethical considerations, existing limitations, the options available, earlier studies, and, above all, focusing on the welfare of laboratory species to fully recognize their enormous contributions to science.
6. Conclusions
Animal models—including a broad diversity of species of vertebrates and invertebrates—are a key element for experimental research aimed at replicating human and animal pathologies. Over the past five years, significant advances regarding worldwide priority diseases such as COVID-19, breast cancer, diabetes, obesity, and Parkinson, among others, were made in species such as nonhuman primates, rodents, lagomorphs, dogs, pigs, and even invertebrates such as zebrafish and nematodes. Moreover, before human clinical trials, novel therapeutic drugs, diagnostic techniques, and surgical procedures such as flaps or organ transplants have also been refined in animals.
These examples show the importance of using animals in biomedical research to study emerging or poorly understood human and animal diseases, and development of novel therapeutic options, including nanoparticles and in vivo techniques. Although animals will remain an essential element of science in the near future, due to their remarkable contributions, the ethical aspect of animal experimentation is significant.
The ethical pressure and the application of initiatives to reduce and replace the number of animals used in experimental protocols is leading to new strategies such as genetic engineering, artificial intelligence, organs-on-chips, mathematical models, bioprinting of organs, and advanced machine learning technologies. This multimodal approach is considered the best option for addressing the ethical dilemmas raised by using laboratory animals while emphasizing their valuable contributions to human and animal medicine.
Funding Statement
This research received no external funding.
Author Contributions
All the authors contributed to the conceptualization, writing, reading, and approval of the final manuscript. All authors have read and agreed to the published version of the manuscript.

Institutional Review Board Statement
Informed consent statement, data availability statement, conflicts of interest.
The authors declare no conflict of interest.
Disclaimer/Publisher’s Note: The statements, opinions and data contained in all publications are solely those of the individual author(s) and contributor(s) and not of MDPI and/or the editor(s). MDPI and/or the editor(s) disclaim responsibility for any injury to people or property resulting from any ideas, methods, instructions or products referred to in the content.
- Search UNH.edu
- Search College of Life Sciences and Agriculture
Commonly Searched Items:
- Academic Calendar
- Programs of Study
- Scholarships
- Study Abroad
- Accelerated Master's Degrees
- Undergraduate Advising
- Pre-Professional Health Advising
- Thompson School of Applied Science
- Department of Agriculture, Nutrition, and Food Systems
- Department of Biological Sciences
- Department of Molecular, Cellular, and Biomedical Sciences
- Department of Natural Resources and the Environment
- Studying Sustainability
- Student Resources
- Employer Resources
- Parents & Families
- COLSA's SOAR Fund
- Meet The Staff
- COLSA Research Directory
- Facilities and Resources
- Hamel Center Research Opportunities
- Map: Research & Partnerships Around the Globe
- NH Agricultural Experiment Station
- Research Centers
- Undergrad Research Resources
- UNH Collections
- Faculty Directory
- Staff Directory
- Faculty and Staff Awards
- Cooperative Extension
- New Hampshire Veterinary Diagnostic Laboratory
- Office of Woodlands and Natural Areas
- UNH Water Quality Analysis Laboratory
- Dean's Office
- Dean's Welcome
- Mission Statement
- Give to COLSA
- Faculty & Staff Resources
- INSPIRED Research Report
- Dean's Awards
- Shiva and Elizabeth Nanda Award for Innovation
- Teaching and Research Scholars Award
- COLSA Newsroom
What Can I Do with a Biomedical Science Degree?
With a biomedical sciences degree, your career possibilities are diverse and abundant. At UNH COLSA , our three Biomedical Science majors are designed to equip you for a variety of roles in the health sector and beyond. You can pursue traditional paths such as research and clinical applications, where you can contribute to advancing medical knowledge and patient care. But your expertise can also be invaluable in less conventional roles that rely on a deep understanding of biology and medicine, such as in science communication, policy making, or health education. In this blog post, we'll be going over the myriad career opportunities a Biomedical Science degree offers you, as well as some tips to help you when you're ready to enter the job market after graduation.
Understanding Biomedical Science
The skills you develop throughout your biomedical sciences education, like analytical thinking and problem-solving, are highly sought after in many fields. For example, roles in pharmaceuticals, biotechnology, and regulatory affairs offer opportunities to apply your knowledge in a corporate or industry setting. Alternatively, your degree can serve as a stepping stone to further education and specialization in fields such as medicine, dentistry, or academia. Each career path appreciates the critical thinking and rigorous scientific training inherent to a biomedical sciences background.
Moreover, the landscape of biomedical sciences careers is always evolving. When new challenges arise, such as public health issues and technological advancements, your degree positions you to be at the forefront of innovation and change. Whether you choose to focus on research, patient care, or industry, you can be confident that a biomedical sciences degree provides a strong foundation for a meaningful and dynamic career.
Scope of Biomedical Sciences
The biomedical sciences encompass an interdisciplinary approach focused on the understanding of human health and disease. As a biomedical science major, you're exposed to a plethora of subjects including biology, biochemistry, cell biology, genetics, immunology, and molecular biology. You might start at the University of New Hampshire (UNH) COLSA, where specialized programs like the Medical Laboratory Science option provide a firm foundation in diagnostic laboratory work, which is crucial in disease treatment and prevention.
Moreover, the biomedical science realm offers diverse opportunities. By pursuing an undergraduate major in this area, you're preparing not just for laboratory-based roles but also a wide range of career options in research, academia, healthcare policy, or pharmaceutical development.
Core Principles and Skills
Biomedical science is grounded in a set of core principles that include understanding the molecular mechanisms of disease, the body's immune responses, and the intricate details of genetic inheritance. Critical thinking and data analysis are central to this discipline, enabling you to decipher complex scientific data and draw meaningful conclusions.
In a program like the Medical and Veterinary Sciences option , you not only delve into biochemistry and molecular biology but also develop communication skills that are essential for conveying scientific information. Whether you’re working on groundbreaking research or communicating your findings, these skills place you at the core of scientific advancements that shape our understanding of life sciences.
Career Paths in Biomedical Science
With a biomedical science degree, you embark on a journey through a versatile field that offers various fulfilling career opportunities tailored to your interests. Whether it's research, technology, or working directly with patients, your choice impacts health outcomes and scientific advancements.
Research and Development
In Research and Development (R&D), you craft the future of medical treatments and health care technologies. As a research scientist or biotechnologist, you may secure funding through organizations like the National Science Foundation (NSF) for groundbreaking projects. Engaging in pharmacology, neuroscience, or other specializations, biomedical science jobs in R&D deliver competitive salaries, reflecting the significant impact of your work.
Clinical and Laboratory Work
Clinical and laboratory work forms the backbone of patient diagnosis and research validity. Working in hospitals or laboratories, your role as a clinical scientist, laboratory technician, or microbiologist contributes to advancements in health. The National Institutes of Health ( NIH ) often supports such critical roles, ensuring that those on the forefront of medical testing and diagnostics have the resources they need.
Biomedical Technology and Engineering
The field of biomedical technology and engineering merges engineering principles with biological knowledge to develop life-saving devices and equipment. As a biomedical engineer, you may work in development, testing, or maintenance, ensuring that technological applications meet the complex needs of medical professionals and patients. This sector often requires collaboration with institutions like the Medical Research Council to align engineering innovation with medical research goals.
Medical Sales and Technical Support
Medical sales and technical support serve as critical links between manufacturers and healthcare providers. A medical sales representative informs and distributes the latest biomedical products, while technical support specialists ensure these technologies function correctly in real-world settings. Your expertise not only drives sales but also supports medical staff, drastically improving patient care and the industry's operational efficiency.
In these dynamic roles, salaries often reflect both your experience and the specialized knowledge required. Whether contributing to cutting-edge medical research or supporting veterinary sciences, a career in biomedical science adapts to your professional aspirations. Those drawn to animal health can explore opportunities through organizations like the American Veterinary Medical Association (AVMA), which empowers professionals in veterinarian roles to excel in their field.
Working in Healthcare
With a biomedical science degree, you're uniquely positioned to play an integral role in advancing healthcare. You can contribute significantly to medicine, health, and safety in various settings.
Hospitals and Clinics
In hospitals and clinics, you can bring value as a clinical research associate or physician associate, working alongside doctors to diagnose and manage health problems. Your expertise supports the effective treatment of diseases, including cancer and chronic conditions linked to aging. Biomedical scientists are also instrumental in the implementation of clinical trials, ensuring new treatments are safe and effective for patient well-being.
Public Health and Safety
With your expertise in biomedical science, your role in public health and safety can pivotal. For example, by understanding and applying principles of epidemiology, you help control health hazards and safeguard communities.
Medical Research
In medical research, your degree enables you to investigate the causes and progression of diseases. You'll play a key role in discovering new interventions to enhance public health. Through research on pressing challenges like cancer and aging, you can contribute significantly to our collective understanding and our ability to improve patient care and wellbeing.
Specialized Fields of Study
With a biomedical science degree, your expertise can significantly contribute to specialized fields. Each one offers a distinct impact on scientific discovery and public health.
Forensic Science
In Forensic Science, you become an integral part of legal investigations. Your role can range from a forensic scientist analyzing evidence to a crime scene investigator meticulously collecting samples onsite. Mastery in this area often requires an in-depth understanding of biological samples and attention to detail.
Genetic Counseling
Genetic Counseling is another path where communication and compassion are as crucial as scientific knowledge. As a genetic counsellor , you'll guide patients through complex genetic information, helping them understand their health risks and options.
Toxicology and Pathology
Moving into Toxicology and Pathology, your work could lead to important safety assessments as a toxicologist, or you might diagnose diseases in a pathology lab as a pathologist. These roles are pivotal in discovering how substances affect living organisms and the causes behind illnesses, respectively.
Roles Beyond the Laboratory
With a biomedical science degree, your career path is not confined to the traditional laboratory environment. You can thrive in various roles that leverage your scientific expertise while exploring realms such as communication, education, and industry.
Science Communication and Journalism
If you possess a knack for translating complex scientific concepts into digestible content, careers in science communication and journalism may suit you. You could become a science writer or science journalist, creating content for publishing companies or media outlets. This role involves researching scientific advancements and conveying them clearly to inform or educate the public.
Education and Teaching
In the field of education, your knowledge can inspire the next generation of scientists. You could pursue a role as a teacher, specializing in STEM teaching, or work as a teaching laboratory technician. These positions allow you to contribute to the scientific literacy of your community and play a direct role in shaping future professionals in the STEM fields.
Industry and Regulatory Roles
There's a continual demand for biomedical science graduates in the pharmaceutical and biotechnology sectors, where you could work as a biotechnologist, developing new products or improving existing ones. Alternatively, regulatory affairs offer positions where you ensure that companies comply with all of the regulations and laws pertaining to their business. These roles require a deep understanding of scientific principles and the ability to apply them within a regulatory framework, balancing scientific innovation with public safety and ethical considerations.
Navigating the Job Market
Entering the job market with a biomedical science degree opens a diversity of doors in various sectors, including academia, industry, and healthcare. Knowing how to present yourself and continuing to develop your skill set are crucial steps in securing your desired role.
Building a Strong Resume
Your resume is often the first impression you make on potential employers. Highlighting your project management and research undertaken at universities or research institutes is vital. Make use of UNH’s resources for templates and how-to guides tailored for crafting resumes that stand out in the biomedical field.
- Education: Include your biomedical science degree and relevant coursework.
- Experience: List internships, work experience, and project involvement, emphasizing any leadership or collaboration roles.
The Importance of Networking
Networking is a key component for biomedical professionals. Engaging with professional associations and attending events hosted by universities can lead to invaluable connections and insight into career opportunities.
- Connect with peers and faculty at your university.
- Reach out to alumni through your university's career services for guidance or mentorship.
Continuing Education and Training
The biomedical field is constantly evolving, making continuous learning essential. Pursue further education, certifications, or training to enhance your marketability as a medical science liaison or other specialized roles.
- Seek continuing education programs to stay current with biomedical advancements.
- Consider certifications in project management to boost your leadership qualifications.
Want to find out more about COLSA? Check us out on:
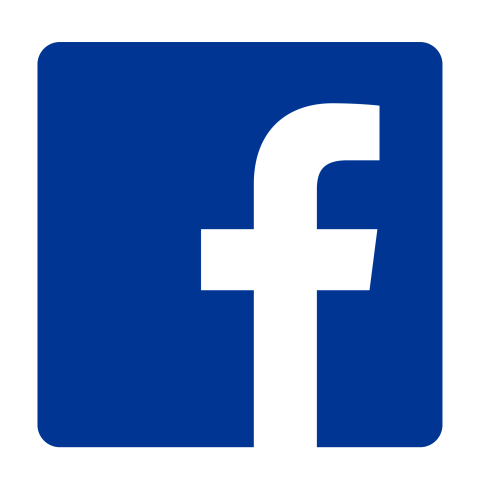
Explore COLSA at UNH
College of life sciences and agriculture.
- Why UNH-in-Italy?
- Admissions & Application
- Code of Conduct
- Health & Well Being
- Costs & Inclusions
- Program Dates
- Join Our Mailing List
- Test duplicate redirects
- Upcoming Events & Info Sessions
- Register with PreHealth Office
- Choosing A Major
- Courses, Grades, and Credits
- Developing an Academic Plan
- Developing Your Health Professions Profile
- Academic Resources
- Explore the Health Professions
- General Outline
- New Applicant Cycle
- Reapplicant Cycle
- Sample Questions
- Study Resources
- Delay Your Application
- Application Outcomes
- Alumni and Post-Bacc Application
- Advisory Committee
- High School Preparation
- Common Questions
- International Students
- Experiential Opportunities
- Get Involved
- Community and Environmental Planning Careers
- Applied Animal Science Careers
- Biochemistry, Molecular and Cellular Biology Careers
- Biology Careers
- Biomedical Science Careers
- Environmental Conservation and Sustainability Careers
- Environmental Sciences Careers
- Environmental and Resource Economics Careers
- Equine Science Careers
- Forestry Careers
- Genetics Careers
- Marine, Estuarine and Freshwater Biology Careers
- Neuroscience and Behavior Careers
- Nutrition Careers
- Sustainable Agriculture and Food Systems Careers
- Veterinary Technology Careers
- Wildlife and Conservation Biology Careers
- Zoology Careers
- Helpful Career Links
- Internship and Job Search Tips
- UNH Career and Professional Success
- Participate In Events
- Why Hire a Wildcat?
- SOAR Application
- Current Projects
- Education & Research
- Course, Research, or Activity (CRA) Registration Form
- Harvest Information
- Bear Camp River
- Burley-Demeritt
- Burnham Lot
- College Woods
- Davis-White Lot
- East Foss Farm
- Horticulture Farm
- Jones Property
- Lovell River
- MacDonald Lot
- Madison Lot
- Mendums Pond
- Moore Fields
- Saddleback Mt.
- Systems Field
- Thompson Farm
- West Foss Farm
- Development Board

- Sustainability
- Embrace New Hampshire
- University News
- The Future of UNH
- Campus Locations
- Calendars & Events
- Directories
- Facts & Figures
- Academic Advising
- Colleges & Schools
- Degrees & Programs
- Undeclared Students
- Course Search
- Career Services
- How to Apply
- Visit Campus
- Undergraduate Admissions
- Costs & Financial Aid
- Net Price Calculator
- Graduate Admissions
- UNH Franklin Pierce School of Law
- Housing & Residential Life
- Clubs & Organizations
- New Student Programs
- Student Support
- Fitness & Recreation
- Student Union
- Health & Wellness
- Student Life Leadership
- Sport Clubs
- UNH Wildcats
- Intramural Sports
- Campus Recreation
- Centers & Institutes
- Undergraduate Research
- Research Office
- Graduate Research
- FindScholars@UNH
- Business Partnerships with UNH
- Professional Development & Continuing Education
- Research and Technology at UNH
- Current Students
- Faculty & Staff
- Alumni & Friends
- Request Information
Skip to content
Explore CUIMC
Diversity, equity, and inclusion.
At CUIMC, we are committed to continuous improvement in providing culturally inclusive medical education and clinical care.
Columbia Vagelos College of Physicians and Surgeons is dedicated to developing the next generation of leaders in medicine
Patient Care
- Find a Doctor
Search for a provider by specialty, expertise, location and insurance. Schedule an appointment online.
Read the latest news stories about CUIMC faculty, research, and events
Columbia University Begins Construction On New York City’s First All-Electric Biomedical Research Building
New building combines state-of-the-art medical research with a vision for a more sustainable future for science, share this page.
- Share on Facebook
- Share on X (formerly Twitter)
- Share on LinkedIn
- Share by email
Columbia University Vagelos College of Physicians and Surgeons (VP&S) will begin construction on New York City’s first all-electric university research building in May. The new biomedical research building in Washington Heights is designed by Kohn Pedersen Fox (KPF) and will house eight stories of laboratories and research facilities, collaboration corners, living walls, and community engagement spaces.
The new biomedical research building will become the center of Columbia’s efforts to gain new understanding of diseases and develop next-generation treatments for some of the most significant threats to human health, including neurodegenerative disease, autoimmune disease, metabolic disorders, heart disease, and cancer.
This will be the first university-owned research building in New York City that does not rely on fossil fuels and fully incorporates sustainability goals into all aspects of its design and operations. The new building will use significantly less energy than similar buildings of its kind and is expected to perform 30% more efficiently than the ASHRAE 90.1 2010 standard, an energy efficiency benchmark for commercial buildings in the United States.
Rendering of Columbia University Vagelos College of Physicians and Surgeons’ new biomedical research building (Credit: Kohn Pedersen Fox)
“We are so proud to be laying the groundwork for this innovative new research building at Columbia. To create a space that will advance biomedical science, bring us closer to our local community, and help our medical center reduce its carbon footprint all in one is truly remarkable,” says Katrina Armstrong, MD , d ean of the Faculties of Health Sciences and the Vagelos College of Physicians and Surgeons and e xecutive vice president for Health and Biomedical Sciences, Columbia University. “ Our purpose as a university is to drive discovery, educate next-generation leaders, and create inclusive partnerships with our community. This new space will offer the best environment for our people to do all three.”
Credit: Kohn Pedersen Fox
The building’s laboratory floors will house 32 principal investigators and their teams of research technicians, postdoctoral researchers, and graduate students. The building will feature unique collaboration corners between research spaces that will help facilitate spontaneous interactions and idea-sharing among scientists. Biophilic elements such as green walls of living plants and the extensive use of natural, renewable materials will help reduce work fatigue and provide health and environmental benefits. The new building is accessible to community partners, providing ground level space to support community health engagement and research education and dissemination activities.
Research laboratories typically consume five to 10 times more energy per square foot than an average office building, according to the Department of Energy. At a time when scientists are increasingly concerned about the environmental impact of their research, Columbia’s new biomedical research building will set a leading example of a more sustainable future for science. To limit energy consumption, research spaces will incorporate many sustainable design strategies including high-efficiency lab fume hoods, demand-based controls for lab equipment, and air-source electric heat pumps.
The new biomedical building will outperform emission limits set by New York City’s Local Law 97 and help advance Columbia University’s Plan 2030 climate goal of achieving campus-wide net-zero emissions by 2050.
Columbia’s new biomedical research building is the latest in a series of climate initiatives by Columbia University, which includes the establishment of the Climate School in 2020 and launch of a global program to incorporate climate change into medical education.
- Subscribe Now (Opens in new window)
- Air Force Times (Opens in new window)
- Army Times (Opens in new window)
- Marine Corps Times (Opens in new window)
- Pentagon & Congress
- Defense News (Opens in new window)
- Flashpoints
- Benefits Guide (Opens in new window)
- Military Pay Center
- Military Retirement
- Military Benefits
- VA Loan Center (Opens in new window)
- Discount Depot
- GearScout (Opens in new window)
- Military Culture
- Military Fitness
- Military Movies & Video Games
- Military Sports
- Transition Guide (Opens in new window)
- Pay It Forward (Opens in new window)
- Military History
- Black Military History (Opens in new window)
- Congressional Veterans Caucus (Opens in new window)
- Military Appreciation Month (Opens in new window)
- Vietnam Vets & Rolling Thunder (Opens in new window)
- Hall of Valor (Opens in new window)
- Service Members of the Year (Opens in new window)
- Create an Obituary (Opens in new window)
- Medals & Misfires
- Installation Guide (Opens in new window)
- Battle Bracket
- CFC Givers Guide
- Task Force Violent
- Newsletters (Opens in new window)
- Early Bird Brief
- Photo Galleries
- Long-Term Care Partners
- Navy Federal
- Digital Edition (Opens in new window)
Navy fires commander of biomedical research lab
:quality(70)/cloudfront-us-east-1.images.arcpublishing.com/archetype/HRKYBOXCT5GZNAT3TMIEQP466U.png)
The Navy fired the commanding officer of a Lima, Peru, based biomedical research lab on Friday, less than a year after she assumed command.
Capt. Abigail Y. Marter was relieved as head of Naval Medical Research Unit South “due to a loss of confidence in her ability to command,” the Navy said in a statement.
Such boilerplate language is often used by the Navy when first announcing the relief of commanding officers and other senior personnel.
Officials did not immediately respond to follow-up questions from Navy Times regarding the reasons for Marter’s firing.
“Navy commanding officers are held to the highest standards of personal and professional conduct,” the Navy said. “They are expected to uphold the highest standards of responsibility, reliability, and leadership, and the Navy holds them accountable when they fall short of meeting these standards.”
Cmdr. Michael Prouty has assumed temporary command of the unit, and Marter has been temporarily reassigned to Naval Medical Research Command.
A family nurse practitioner, Marter took command of the unit in July.
Formerly known as Naval Medical Research Unit 6, the command monitors and researches infectious diseases in Central and South America.
Its main hub is on a Peruvian naval base, but the command also runs a satellite lab on an air base in Honduras.
Geoff is the editor of Navy Times, but he still loves writing stories. He covered Iraq and Afghanistan extensively and was a reporter at the Chicago Tribune. He welcomes any and all kinds of tips at [email protected].
In Other News
:quality(70)/cloudfront-us-east-1.images.arcpublishing.com/archetype/XHCUSJLAJVBIJBGQRFK3AFPZCI.png)
Trial begins for Navy chief facing multiple espionage charges
Chief fire controlman (aegis) bryce steven pedicini is the first sailor to face espionage charges within the navy justice system in at least five years..
:quality(70)/cloudfront-us-east-1.images.arcpublishing.com/archetype/AZ3ZTBQDZVAGJKTASXHY3K5IB4.jpg)
US doesn’t want to make Arctic contested battlespace, admiral says
“there’s no desire to over-militarize or create a theme of a contested battlespace in the arctic," the commander of u.s. 2nd fleet said..
:quality(70)/cloudfront-us-east-1.images.arcpublishing.com/archetype/VCIH6X4C5FHFPFJ6I4NG43OVKU.jpg)
The salty sailors of the warship USS Carney have left the Middle East
Where the battle-tested carney crew is heading next remains unclear, as officials declined to say if the warship is heading home..
:quality(70)/cloudfront-us-east-1.images.arcpublishing.com/archetype/6ZKJ6WX4SFEVVK7X7PFR2ZLZMU.jpg)
Lawmakers push for cost-of-living boost in veterans benefits next year
Legislation introduced in the senate would guarantee a cost-of-living boost in veterans benefits in 2025..
:quality(70)/cloudfront-us-east-1.images.arcpublishing.com/archetype/G4M3PHUBYVE6NFR22BKY3VUFEQ.jpg)
Military pharmacies resume regular operations after cyberattack
Military pharmacies no longer have to manually process prescriptions following a february cyberattack that disrupted pharmacy operations around the world..

COMMENTS
Biomedical Research synonyms - 115 Words and Phrases for Biomedical Research. biomedical science. animal experimental. animals used for experiments. bio medical research. bio-medical. bio-medicine. bio-research. bioanalysis.
Such is the case for the term "translational research," which is defined by the European Society of Translational Medicine as an interdisciplinary branch of biomedical science supported by 3 main pillars: benchside, bedside, and community ( 1 ). Defined in this way, translational research involves the application of scientific observations ...
However, previous research has not established either the best embedding methods for detecting synonyms among related word pairs or how effective such methods may be. ... We observed 2 problems using cosine similarity for identifying synonyms for a biomedical search. The first is the absence of consensus regarding the embedding method that ...
Algorithms. Biomedical Research*. Information Storage and Retrieval / methods*. Linguistics*. Probability. PubMed*. Terminology as Topic. We introduced the BioSearchSyn corpus of 1000 term pairs, which allowed us to identify the best embedding method for detecting synonymy for biomedical search. Using the proposed method, we created ...
Basic biomedical research, which addresses mechanisms that underlie the formation and function of living organisms, ranging from the study of single molecules to complex integrated functions of humans, contributes profoundly to our knowledge of how disease, trauma, or genetic defects alter normal physiological and behavioral processes. Recent advances in molecular biology techniques and ...
Utilizing biotechnology techniques, biomedical researchers study biological processes and diseases with the ultimate goal of developing effective treatments and cures. Biomedical research is an evolutionary process requiring careful experimentation by many scientists, including biologists and chemists. Discovery of new medicines and therapies ...
Best synonyms for 'biomedical research' are 'bio medical research', 'biological research' and 'biomedical researches'.
Biomedical Research. Few topics in biomedical research, other than research funding and the JIF, the latter of which is a direct output of the peer review process, have galvanized opinion in biomedical researchers to the same degree as peer review with numerous erudite and often emotive statements that reflect on the innate bias of reviewers and editors, inconsistencies in reviewing standards ...
Biomedical research applies the principles of the physical sciences to medicine. Most biomedical research is conducted by physicians or biomedical scientists, but many studies are conducted by biologists, chemists, physicists, and other medical and scientific professionals. Most biomedical research involves clinical trials, which are phased ...
Biomedical Synonyms. Meanings Synonyms Sentences Common Words Unique Words. Words Related to Biomedical Related words are words that are directly connected to each other through their meaning, even if they are not synonyms or antonyms. ... But the only European biomedical research that has used great apes recently is the Biomedical Primate ...
3. Noun Usage: Biomedical research can also function as a noun in a sentence, typically referring to the overall field or specific studies. Here are a couple of examples: "Biomedical research plays a crucial role in understanding complex diseases." "The findings of this biomedical research have significant implications for public health."
BIOMEDICAL meaning: 1. relating to biology and medicine: 2. relating to biology and medicine: 3. used to describe…. Learn more.
Here's a list of similar words from our thesaurus that you can use instead. Adjective. Relating to biology and medicine. biological. medical. health-related. "The professor specialized in biomedical research, exploring the intersection between biology and medicine to develop new treatments for diseases.". Find more words!
The field of biomedical research continues to push the boundaries of what is possible in healthcare. The advancements in precision medicine, immunotherapy, biomaterials and tissue engineering, AI ...
Synonyms for biomedical research bio·med·ical re·search This thesaurus page includes all potential synonyms, words with the same meaning and similar terms for the word biomedical research. We couldn't find direct synonyms for the term biomedical research. Maybe you were looking for one of these terms?
An antonym for "ignominious". A opprobrious. B smuggled. C inglorious. D honorable. Find all the synonyms and alternative words for biomedical at Synonyms.com, the largest free online thesaurus, antonyms, definitions and translations resource on the web.
1. Introduction. The use of animals in scientific research is controversial [].However, the transformation of medicine from an art to a science can be mainly attributed to using a wide range of animal models [], selected according to their functional and genetic characteristics for specific research lines [].Animal models contribute significantly to the advance of biomedical science through ...
Pros. • Biomedical research is promising cause area. • The field seems to be constrained by good researchers. • Highly interesting work for the intellectually curious. Cons. • Long time to train (4-12 years). • Highly competitive; people drop out even in their late thirties and forties.
In Research and Development (R&D), you craft the future of medical treatments and health care technologies. As a research scientist or biotechnologist, you may secure funding through organizations like the National Science Foundation (NSF) for groundbreaking projects. Engaging in pharmacology, neuroscience, or other specializations, biomedical ...
Columbia's new biomedical research building is the latest in a series of climate initiatives by Columbia University, which includes the establishment of the Climate School in 2020 and launch of a global program to incorporate climate change into medical education. Columbia University Irving Medical Center. NewYork-Presbyterian.
Apr 5, 01:47 PM. Capt. Abigail Y. Marter was relieved of command of Naval Medical Research Unit South on Friday. (Navy) The Navy fired the commanding officer of a Lima, Peru, based biomedical ...